Cuproptosis, the novel therapeutic mechanism for heart failure: a narrative review
Introduction
Heart failure (HF) is the terminal stage of various heart diseases, and is caused by systolic and/or diastolic dysfunction of the heart, which may be impaired for various reasons. Although a large number of clinical studies and new drug trials have been carried out for HF, the rehospitalization rate and mortality rate are still high (1). Currently, immunity, inflammation, and oxidative stress are considered to be important pathophysiological processes in HF and may serve as emerging therapeutic targets for treating this condition (2). Mitochondria have become an important therapeutic target for HF due to their critical roles in cardiac energy supply, oxidative stress, calcium regulation, and inflammatory mechanisms (3-5). Regardless of etiology, mitochondrial dysfunction in HF is widespread.
The micronutrient copper plays an important role in the maintenance of human body homeostasis and has antioxidant properties (6). Research has found that copper has unique effects on the mitochondrial electron transfer chain (mtETC), tricarboxylic acid (TCA) cycle, and reactive oxygen species (ROS) production (7,8). Both superoxide dismutase 1 (Sod1) and cytochrome c oxidase (CcO) in mitochondria require the participation of copper. Copper is closely related to the pathophysiological process of the cardiovascular system (9), and high copper concentrations are significantly associated with HF and its prognosis (10-13). A study of rats with HF found that copper chelation and the restoration of myocellular copper trafficking could repair mitochondria and improve cardiac function (14). However, the mechanism underlying the relationship between HF and copper overloading remains unclear.
The latest research has discovered a unique cell death mechanism, cuproptosis, which differs from apoptosis, pyroptosis, and ferroptosis (15). Specifically, the regulation of cuproptosis is based on copper overload, is dependent on mitochondrial respiration and adenosine triphosphate (ATP) production, and is closely related to the TCA cycle. Therefore, we rationally hypothesize that cuproptosis could be a novel therapeutic target for HF, through the regulation of mitochondria. Because copper concentrations are generally elevated in studies of HF, and the regulation of cuproptosis is based on copper overload, the effect of copper deficiency on HF is not discussed here. We present the following article in accordance with the Narrative Review reporting checklist (available at https://cdt.amegroups.com/article/view/10.21037/cdt-22-214/rc).
Methods
The PubMed database was used to perform a literature search up to April 2022, and publications in non-English languages and publications with low credibility were excluded. Specific searches included different packages of the following search terms: “cuproptosis”, “mitochondria”, “heart failure”, “copper”, “zinc”, “selenium”, “FDX1”, “acetylation”. Table 1 shows our search formulas and strategies. After further literature search and screening, we found that we are currently the first study to explore the association between HF and cuproptosis. In addition, Table S1 provides a list of full terms.
Table 1
Items | Specification |
---|---|
Date of search | 22/04/2022 |
Databases and other sources search | PubMed |
Search terms used | “cuproptosis” [MeSH] |
“heart failure” [MeSH] | |
“mitochondria” [MeSH] | |
(“mitochondria” [MeSH]) AND “heart failure” [MeSH] | |
“copper” [MeSH] | |
(“copper” [MeSH]) AND “mitochondria” [MeSH] | |
(“copper” [MeSH]) AND “heart failure” [MeSH] | |
(“zinc” [MeSH]) AND “heart failure” [MeSH] | |
(“selenium” [MeSH]) AND “heart failure” [MeSH] | |
“FDX1” [MeSH] | |
(“acetylation” [MeSH]) AND “heart failure” [MeSH] | |
Timeframe | Up to April 2022 |
Inclusion and exclusion criteria | Inclusion criteria: research articles and reviews about themes such as HF, mitochondria, micronutrient copper and cuproptosis |
Exclusion criteria: non-English publications and publications with low credibility were excluded | |
Selection process | It was conducted independently by HJY, YTX, YL; data selection is the intersection of the search of three authors |
HF, heart failure.
Pathophysiology of mitochondria and HF
The heart is the core of human energy, and most of the ATP (about 95%) it consumes is produced by oxidative metabolism in the mitochondria (5), which occurs on the inner mitochondrial membrane. Reducing equivalents such as nicotinamide adenine dinucleotide (NADH) and flavin adenine dinucleotide (FADH2), produced by various substrates through the TCA cycle, are transferred through the mtETC to generate a proton gradient, and subsequently promote the phosphorylation of adenosine diphosphate (ADP) to form ATP (Figure 1). ROS is also generated during this process (16). In addition, mitochondria are extensively involved in pathophysiological processes (17-19), including energy metabolism, oxidative stress, calcium regulation, cell death, and signaling.
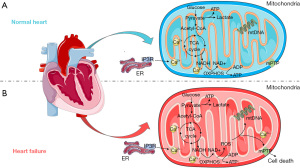
Energy metabolism
Patients with HF are characterized by insufficient cardiac energy metabolism. As HF progresses, mitochondrial oxidative capacity is reduced, and complex energy metabolism changes occur (Figure 1) (20,21). The use of angiotensin-converting enzyme inhibitors (ACEI) can reduce myocardial oxygen consumption and improve cardiac function, while drugs that enhance myocardial oxygen consumption, such as epinephrine, can lead to the worsening of HF. In addition, the ACEI trandolapril has been confirmed to improve mitochondrial function and prevent the development of HF.
Posttranslational modifications (PTMs) are widely involved in the physiological processes of cells and play an important role in the regulation of protein activity and function. Among mitochondrial proteins, the most studied modification is acetylation, which refers to the transfer of the acetyl group of acetyl coenzyme A (acetyl-CoA) to a lysine residue. In particular, pyruvate dehydrogenase (PDH), TCA cycle enzymes, and ETC proteins are highly acetylated (22,23). Increased mitochondrial protein lysine acetylation and decreased levels of mitochondrial deacetylases sirtuin 3 (SIRT3) and NADH/NAD+ ratio have been observed in animal models and patients of HF (22-24). Mitochondrial acetylation alters the NADH/NAD+ redox state and ATP synthesis (23-25). SIRT3 knockout mice have been shown to exhibit enhanced sensitivity to mitochondrial permeability transition pore (mPTP) opening and increased HF mortality (26). The hyperacetylation of mitochondrial proteins is an important influencing factor of energy metabolism disorder in HF. Lipid overload may be responsible for regulating the state of protein acetylation and the function and activity of cardiomyocytes through the reduction of NAD+ and the generation of acetyl-CoA synthesis (27). However, the cause of mitochondrial protein hyperacetylation in HF is unclear.
Generation of ROS and oxidative stress
Mitochondria are the main source of ROS in cardiomyocytes. Under normal circumstances, ROS is only a by-product of the mitochondrial oxidative phosphorylation (OXPHOS) process, but in HF, the level of ROS in mitochondria is significantly increased (28), which can cause oxidative stress and severe cardiac damage, and accelerate the occurrence and development of HF (29). ROS in HF mainly arises from the uncoupling of mtETC, and multiple sites of complexes I and III can partially reduce oxygen to superoxide (30,31), which can be rapidly disproportionated to hydrogen peroxide by Sod. A deficiency of glutathione peroxidase 1 (Gpx1), which removes intracellular hydrogen peroxide, has been linked to enhanced oxidative stress and accelerated cardiac dysfunction (32). Moreover, mitochondrial protein hyperacetylation of HF inhibits the activity of ATP synthase, which not only attenuates ATP production but also enhances ROS release (33).
Mitochondrial ROS (mtROS) causes DNA damage, and extensive oxidation of proteins and lipids and can also trigger multiple cellular pathways and mediate cell death (Figure 1) (34,35). Furthermore, mtROS within cardiomyocytes impairs myocardial function, including ion transport, contractility, and calcium cycling (36). The association of mtROS with inflammation and cardiovascular disease has been widely reported (37-39). Studies support clear cardiac benefits of inhibiting mtROS production and apoptosis in HF (40), while simvastatin has been shown to improve HF by reducing ROS production and inhibiting mitochondria-mediated apoptosis (41).
Calcium regulation
The calcium transport system of mitochondria is very active. Mitochondrial calcium uptake is an important cause of intracellular calcium homeostasis, and calcium can activate various enzymes of the OXPHOS pathway (5,42). The synthesis of mitochondrial ATP and ROS is also related to Ca2+. Mitochondrial calcium regulation is achieved through the sarcoplasmic reticulum (SR) inositol 1,4,5-trisphosphate receptor (IP3R) channel opening and calcium release. In HF, SR calcium leakage impairs calcium reuptake, resulting in mitochondrial calcium overload.
Mitochondrial function is significantly regulated by Ca2+. Mitochondrial calcium regulation is involved in the mitochondrial TCA cycle and OXPHOS processes, including the electronic supply of mtETC and activation of Krebs cycle dehydrogenases, which can maintain a stable supply of cardiac energy (43). Mitochondrial calcium overload disrupts this process, resulting in reduced ATP synthesis and increased ROS release (Figure 1) (44,45). It has been widely established that mitochondrial calcium overload is a key factor in HF (46).
Cell death
The central mediators of cardiomyocyte survival and death are mitochondria, which not only support life activities, but also trigger cell death. Mitochondrial calcium overload and ROS accumulation can trigger the opening of the mPTP, allowing for the passage of ions and solutes, the subsequent loss of mitochondrial membrane potential and decoupling of the ETC, and ultimately mitochondrial rupture (Figure 1) (47-50). Loss of membrane potential and the release of cytochrome c from mitochondria can induce apoptosis, while a dramatic drop in ATP levels leads to necrosis (5,49-53). Furthermore, research indicates that autophagy may also be triggered by mtROS (54), and in one study, blocking midgut autophagy-dependent cell death in Drosophila observed accumulation of mitochondria (55). The mitochondria of cells dying by autophagy contain a large number of electron-bound autophagic cells (56). However, the interaction between the autophagy signaling pathway and mitochondria still needs to be further explored.
Research has found that transplantation of autologous mitochondria can treat right HF by reducing the loss of apoptotic cardiomyocytes and preserving myocardial contractility (57). Although we propose that targeting mitochondria may be an emerging therapy for the treatment of HF, clinical drug experiments such as the phase III trial of the mPTP-inhibitor cyclosporine have not yet achieved breakthrough progress (3). There may exist undetected underlying mechanisms that need to be clarified if mitochondrial-targeted therapy is to be further developed.
Copper-induced cell death and HF
Copper is an essential micronutrient for the human body, and cells maintain copper concentration through active homeostatic mechanisms to avoid intracellular copper accumulation (58,59). Studies have shown that when the concentration of copper exceeds the threshold, it is toxic (60). In HF patients, micronutrient deficiencies such as zinc and selenium are prevalent, but copper concentrations are generally elevated (61,62). Tables 2-4 show the status of copper (12,13,63-68), zinc (13,63-65,69), and selenium (63,69-73) in observational studies of HF patients, respectively. A meta-analysis based on 13 studies (1,504 participants) showed that high serum copper was significantly associated with HF (10), and other research has shown that high copper concentrations are associated with mortality and morbidity in HF (11,66).
Table 2
Country, author, year | Methods and study population | Result |
---|---|---|
Czech Republic, Malek et al., 2006 (12) | A 12-month follow-up of 30 patients with chronic HF with acute decompensation and 30 patients with chronic stable HF | The combined hospital admission or end-point of death was significantly associated with serum copper concentrations in both groups (P<0.0001) |
Turkey, Koşar et al., 2006 (63) | 54 HF patients, aged 62±10 years, 55% male; 30 healthy participants, aged 56±8 years, 60% male | Serum copper levels were significantly higher in HF patients compared to controls (880±185 vs. 644±179 μg/L, P<0.001) |
Iran, Shokrzadeh et al., 2009 (64) | 30 HF patients secondary to ischemic cardiomyopathy, aged 57.17±8.88 years, 53% male; 27 healthy participants, aged 42.3±8.99 years, 45% male | Serum copper levels were significantly higher in HF patients compared to controls (1.54±0.52 vs. 1.31±0.24 mg/L, P=0.048) |
Iran, Ghaemian et al., 2011 (65) | 78 HF patients (including 40 atrial fibrillation patients and 38 sinus rhythm patients) 40 age-matched controls | There was no significant difference in serum copper levels between the atrial fibrillation (72.0±44.3 vs. 77.6±6.4 μg/dL, P=0.676) and the sinus rhythm (66.62±25.0 vs. 77.6±6.4 μg/dL, P=0.233) groups compared with the control group |
Greece, Alexanian et al., 2014 (13) | 125 HF patients (including 81 acute HF patients and 44 chronic HF patients), aged 69±11 years, 71.2% male; 21 healthy participants, aged 57±19 years, 81.0% male | Serum copper levels were significantly higher in acute HF (117.77±35.38 vs. 92.71±16.97 μg/dL, P=0.006) and chronic HF (121.43±33.92 vs. 92.71±16.97 μg/dL, P=0.002) patients compared to controls |
Czech Republic, Málek et al., 2003 (66) | Among 64 patients with chronic HF, 30 died or were hospitalized due to worsening HF occurred during 1-year median follow-up | Mean baseline serum copper concentrations were significantly higher in patients who died or were hospitalized due to worsening HF than those who did not worsen (19.0 vs. 16.3 μmol/L, P<0.001) |
Greece, Chrysochou et al., 2021 (67) | 96 HF patients, age >18 years; 35 gender-matched healthy participants, age >18 years | Serum copper levels were significantly higher in HF patients compared to controls [1.332 mg/L, 1.120 vs. 1.027 mg/L, 1.08 (mean, geometric SD), P<0.001] |
Finland, Kunutsor et al., 2022 (68) | Among 1,866 male patients aged 42–61 years with no history of HF, 365 cases of HF occurred during 26.5 years median follow-up | Increased serum copper levels were significantly associated with HF risk [HR =2.42 (1.32–4.44), P=0.004] |
HF, heart failure; HR, hazard ratio.
Table 3
Country, author, year | Methods and study population | Result |
---|---|---|
France, de Lorgeril et al., 2001 (69) | 21 HF patients 18 sex- and age-matched healthy participants | Serum zinc levels were significantly lower in HF patients compared to controls [0.82 (0.12) vs. 0.90 (0.09) mg/L, P<0.05] |
Turkey, Koşar et al., 2006 (63) | 54 HF patients, aged 62±10 years, 55% male; 30 healthy participants, aged 56±8 years, 60% male | Serum zinc levels were significantly lower in HF patients compared to controls (555±104 vs. 620±130 μg/L, P<0.01) |
Iran, Shokrzadeh et al., 2009 (64) | 30 HF patients secondary to ischemic cardiomyopathy, aged 57.17±8.88 years, 53% male; 27 healthy participants, aged 42.3±8.99 years, 45% male | Serum zinc levels were not significantly different between HF patients and controls (1.05±0.28 vs. 1.12±0.42 mg/L, P=0.42) |
Iran, Ghaemian et al., 2011 (65) | 78 HF patients (including 40 atrial fibrillation patients and 38 sinus rhythm patients); 40 age-matched controls | Serum zinc levels were significantly lower in the atrial fibrillation (23.2±16.8 vs. 70.9±21.6 μg/dL, P<0.001) and sinus rhythm (24.7±27.6 vs. 70.9±21.6 μg/dL, P<0.001) groups compared to the control group |
Greece, Alexanian et al., 2014 (13) | 125 HF patients (including 81 acute HF patients and 44 chronic HF patients), aged 69±11 years, 71.2% male; 21 healthy participants, aged 57±19 years, 81.0% male | Serum zinc levels were significantly reduced in both acute HF (74.27±15.87 vs. (87.90±17.85 μg/dL, P<0.001) and chronic HF (81.51±19.73 vs. 87.90±17.85 μg/dL, P=0.039) patients compared to controls |
HF, heart failure.
Table 4
Country, author, year | Methods and study population | Result |
---|---|---|
France, de Lorgeril et al., 2001 (69) | 21 HF patients 18 sex- and age-matched healthy participants | Serum selenium levels were significantly lower in HF patients compared to controls [62.6 (13.9) vs. 77.9 (10.7) μmol/L, P=0.0004] |
Turkey, Koşar et al., 2006 (63) | 54 HF patients, aged 62±10 years, 55% male; 30 healthy participants, aged 56 ± 8 years, 60% male | Serum selenium levels were significantly lower in HF patients compared to controls (121±5 vs. 147±13 μg/L, P<0.01) |
11 European countries, Bomer et al., 2020 (70) | Among 2,516 HF patients age >18 years, 963 patients died or hospitalized for HF during 21 months median follow-up | Low serum selenium concentrations were significantly associated with mortality and hospitalization in HF [HR =1.23 (1.06–1.42), P=0.007] |
Iran, Mirdamadi et al., 2019 (71) | 32 HF patients, aged 64.6±11.9 years, 71.9% male; 32 controls without HF, aged 62.2±11.3 years, 53.1% male | Serum selenium levels were significantly lower in HF patients compared to controls (92.5±22.44 vs. 109.3±29.62 mg/dL, P=0.013) |
China, Zhang et al., 2021 (72) | Among 411 HF patients with a mean age of 62.5 years, 131 patients died for HF during 23 months median follow-up | Low serum selenium levels significantly associated with increased mortality risk in HF patients [HR =2.32 (1.43–3.77), P=0.001] |
The Netherlands, Al-Mubarak et al., 2022 (73) | Among 5,973 patients with a mean age of 53.6±12.1 years, 194 patients developed HF during a median follow-up period of 8.4 years | High serum selenium levels are independently associated with a reduction in new-onset HF [HR =0.82 (0.69–0.96), P=0.017] |
HF, heart failure; HR, hazard ratio.
The specificity of copper’s transformation between 2 oxidation states leads to its interaction with a variety of oxidoreductases that are involved in important physiological processes; examples of these oxidoreductases include lysyl oxidase, which stabilizes the extracellular matrix, and Sod, which prevents oxidative stress (9). Oxidative stress and mitochondrial function may be disturbed by copper regulation (74). Copper ionophores are sensitive to fatty acid, mitochondrial function inhibitors, and mitochondrial antioxidants (15). The participation of copper is also required for the efficient production of ATP in the mitochondrial OXPHOS process. An excessive of copper can disrupt mtETC function and induce the formation of ROS, thereby generating hydroxyl radicals and impairing antioxidant function (8,75,76). Experiments have shown that copper chelators restore the expression of key mitochondrial biogenesis regulators and improve myocardial function in HF rats (77). The effect of copper on mitochondrial function is concentration-dependent (8), therefore, copper overload may be a key reason for mitochondrial dysfunction in HF. However, there are few studies on copper and HF, and the mechanism between HF and copper overload is still unclear.
Mitochondria are closely related to cell death in HF including necrosis, apoptosis, and autophagic cell death. Recent research has identified cuproptosis, a cell death mechanism distinct from apoptosis, pyroptosis, necroptosis, and ferroptosis (15). Copper-induced cell death is closely related to mitochondrial respiration and ATP production. Copper does not directly target the ETC, but rather the TCA cycle, this is because the study found basal respiration was not significantly diminished, but a reduced reserve capacity for respiration. Moreover, protein lipoylation is required for copper-binding, as copper-binds to lipoylated TCA cycle proteins and mediates the loss of iron-sulfur (Fe-S) cluster proteins, triggering proteotoxic stress and enhances toxicity (Figure 2). Because cuproptosis occurs through copper overload and is closely related to lipoylated TCA cycle proteins, it is reasonable to hypothesize that cuproptosis exists in HF and may be a potential therapeutic mechanism for HF.
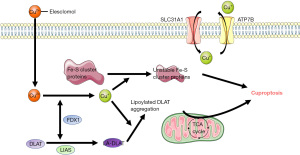
Lipoylation is a lysine PTM, that involves the covalent attachment of lipoyl amide to a lysine residue through an amide bond (78). This is currently known to occur only in dihydrolipoamide branched chain transacylase E2 (DBT), glycine cleavage system protein H (GCSH), dihydrolipoamide S-succinyltransferase (DLST), and dihydrolipoamide S-acetyltransferase (DLAT), which are all important parts of the PDH complex (15). The PDH complex participates in the mitochondrial TCA cycle and OXPHOS process and catalyzes the decarboxylation of pyruvate to acetyl-CoA, which is an important source of acetylation. In addition, the PDH complex contains an E3-binding protein that has a lipoyl domain. Its lipoyl domain undergoes lipoylation, but this lipoylation is thought to be involved in the reduction of acetylation. E2 is also involved in the reduction of acetylation of the complex (79). The binding of copper to the lipoylated components results in a decrease in acetylation reduction in the regulatory mechanism of cuproptosis. This may be the reason for the hyperacetylation of mitochondrial proteins in HF.
Copper chelators are not only used as anticancer agents (80-82), but have also been shown to be closely related to the treatment of HF. Experimental studies have demonstrated that copper chelators restore the expression of peroxisome proliferator-activated receptor gamma coactivator-1α (Pgc-1α), which is a key regulator of mitochondrial biogenesis. Copper chelators can also restore muscle cell copper trafficking and improve heart pump function (14). The copper chelator trientine has entered the drug development stage for the treatment of HF (74), and copper chelators have been shown to partially rescue copper-induced cell death (15). This further confirms the possibility that cuproptosis is a key target of HF.
Furthermore, ferredoxin 1 (FDX1) is closely related to copper-mediated cell death and may be an upstream regulator of protein lipoylation. In one study, FDX1 knockout resulted in a complete loss of protein lipoylation (15). Another study found FDX1 to be involved in fatty acid oxidation, glucose metabolism, and amino acid metabolism and promotes the generation of ATP (83). Copper ionophore has also been shown to cause Fe-S protein loss and further induce proteotoxic stress in an FDX1-dependent manner (15,84,85). However, at present, FDX1 is mainly considered to be closely related to cancer (83,84,86), and its correlation with HF needs further research.
Discussion
Despite great progress in modern medicine, HF is still a major problem in medical care and public health. Readmission rates, mortality, and hospitalization burden have not decreased in patients with HF (87). Thus, there is an urgent need to find novel therapeutic targets and methods for HF. Mitochondria are the main organelle for ATP synthesis in the human body and the main source of energy for the heart. Multiple dysfunctions of mitochondria are key players in the development of HF and have been widely discussed (5,88,89). Mitochondria are a key therapeutic target for HF by virtue of their participation in cardiac energy metabolism, oxidative stress, calcium regulation, and mediating cell death (30,90). However, there has been no breakthrough in drug development targeting mitochondria, possibly because the key underlying mechanisms involved remain unclear.
The micronutrient copper plays an important role in maintaining body homeostasis and is involved in mitochondrial biological processes. In studies of HF, high serum copper was significantly associated with HF (10,11). Copper overload affects mitochondrial function and exacerbates the development of HF. The latest research proposes that cuproptosis, cell death induced by copper overload targeting lipoylated TCA cycle proteins, is closely related to mitochondrial respiration (15). Copper chelators not only partially rescue copper-induced cell death, but also restore Pgc-1α expression and improve cardiac pump function. Therefore, we reasonably hypothesize that cuproptosis may be a novel therapeutic target for HF. Copper binding to lipoylated components may be the reason for the hyperacetylation of mitochondrial proteins in HF.
Experiments found that deletion of FDX1 causes resistance to copper-induced cell death. Moreover, FDX1 is highly correlated with the expression of lipoylated proteins, and knockout of FDX1 leads to complete loss of protein lipoylation (15). Therefore, we hypothesize that FDX1 may be a key target for the treatment of HF based on cuproptosis, which may regulate mitochondrial protein hyperacetylation and reduce cell death in HF. However, current research on FDX1 is still limited to its application in cancer, and the correlation with HF needs further experimental and clinical studies. Furthermore, experiments have found that copper chelators partially rescue copper-induced cell death (15), and copper chelators have been shown to be closely related to the treatment of HF (14,74). Therefore, we suggest that incorporating the cuproptosis mechanism may contribute to the further development of copper chelators as anti-HF drugs.
In conclusion, cuproptosis may be a novel therapeutic mechanism for HF and may be the reason for mitochondrial protein hyperacetylation in HF. This paper provides a new research direction for the treatment of HF and new ideas for the development of new drugs. Furthermore, whether through cuproptosis, necrosis, or apoptosis, mitochondria play a central role in the mechanism of cell death and the occurrence and development of HF.
Acknowledgments
The authors would like to acknowledge C. Gourlay and J. Gray (AME Editing Service) for their assistance with language editing.
Funding: This research was supported by the National Nature Science Foundation of China (No. 81774247).
Footnote
Reporting Checklist: The authors have completed the Narrative Review reporting checklist. Available at https://cdt.amegroups.com/article/view/10.21037/cdt-22-214/rc
Conflicts of Interest: All authors have completed the ICMJE uniform disclosure form (available at https://cdt.amegroups.com/article/view/10.21037/cdt-22-214/coif). The authors have no conflicts of interest to declare.
Ethical Statement: The authors are accountable for all aspects of the work in ensuring that questions related to the accuracy or integrity of any part of the work are appropriately investigated and resolved.
Open Access Statement: This is an Open Access article distributed in accordance with the Creative Commons Attribution-NonCommercial-NoDerivs 4.0 International License (CC BY-NC-ND 4.0), which permits the non-commercial replication and distribution of the article with the strict proviso that no changes or edits are made and the original work is properly cited (including links to both the formal publication through the relevant DOI and the license). See: https://creativecommons.org/licenses/by-nc-nd/4.0/.
References
- Patel SR, Piña IL. From acute decompensated to chronic heart failure. Am J Cardiol 2014;114:1923-9. [Crossref] [PubMed]
- Ayoub KF, Pothineni NVK, Rutland J, et al. Immunity, Inflammation, and Oxidative Stress in Heart Failure: Emerging Molecular Targets. Cardiovasc Drugs Ther 2017;31:593-608. [Crossref] [PubMed]
- Brown DA, Perry JB, Allen ME, et al. Expert consensus document: Mitochondrial function as a therapeutic target in heart failure. Nat Rev Cardiol 2017;14:238-50. [Crossref] [PubMed]
- Kiyuna LA, Albuquerque RPE, Chen CH, et al. Targeting mitochondrial dysfunction and oxidative stress in heart failure: Challenges and opportunities. Free Radic Biol Med 2018;129:155-68. [Crossref] [PubMed]
- Zhou B, Tian R. Mitochondrial dysfunction in pathophysiology of heart failure. J Clin Invest 2018;128:3716-26. [Crossref] [PubMed]
- Uriu-Adams JY, Keen CL. Copper, oxidative stress, and human health. Mol Aspects Med 2005;26:268-98. [Crossref] [PubMed]
- Sappal R, Macdougald M, Stevens D, et al. Copper alters the effect of temperature on mitochondrial bioenergetics in rainbow trout, Oncorhynchus mykiss. Arch Environ Contam Toxicol 2014;66:430-40. [Crossref] [PubMed]
- Isei MO, Kamunde C. Effects of copper and temperature on heart mitochondrial hydrogen peroxide production. Free Radic Biol Med 2020;147:114-28. [Crossref] [PubMed]
- Fukai T, Ushio-Fukai M, Kaplan JH. Copper transporters and copper chaperones: roles in cardiovascular physiology and disease. Am J Physiol Cell Physiol 2018;315:C186-201. [Crossref] [PubMed]
- Huang L, Shen R, Huang L, et al. Association between serum copper and heart failure: a meta-analysis. Asia Pac J Clin Nutr 2019;28:761-9. [PubMed]
- Grammer TB, Kleber ME, Silbernagel G, et al. Copper, ceruloplasmin, and long-term cardiovascular and total mortality (the Ludwigshafen Risk and Cardiovascular Health Study). Free Radic Res 2014;48:706-15. [Crossref] [PubMed]
- Malek F, Jiresova E, Dohnalova A, et al. Serum copper as a marker of inflammation in prediction of short term outcome in high risk patients with chronic heart failure. Int J Cardiol 2006;113:e51-3. [Crossref] [PubMed]
- Alexanian I, Parissis J, Farmakis D, et al. Clinical and echocardiographic correlates of serum copper and zinc in acute and chronic heart failure. Clin Res Cardiol 2014;103:938-49. [Crossref] [PubMed]
- Zhang S, Liu H, Amarsingh GV, et al. Restoration of myocellular copper-trafficking proteins and mitochondrial copper enzymes repairs cardiac function in rats with diabetes-evoked heart failure. Metallomics 2020;12:259-72. [Crossref] [PubMed]
- Tsvetkov P, Coy S, Petrova B, et al. Copper induces cell death by targeting lipoylated TCA cycle proteins. Science 2022;375:1254-61. [Crossref] [PubMed]
- Wang Y, Li N, Zhang X, et al. Mitochondrial metabolism regulates macrophage biology. J Biol Chem 2021;297:100904. [Crossref] [PubMed]
- Gong Y, Lin J, Ma Z, et al. Mitochondria-associated membrane-modulated Ca2+ transfer: A potential treatment target in cardiac ischemia reperfusion injury and heart failure. Life Sci 2021;278:119511. [Crossref] [PubMed]
- Pfanner N, Warscheid B, Wiedemann N. Mitochondrial proteins: from biogenesis to functional networks. Nat Rev Mol Cell Biol 2019;20:267-84. [Crossref] [PubMed]
- Chipuk JE, Mohammed JN, Gelles JD, et al. Mechanistic connections between mitochondrial biology and regulated cell death. Dev Cell 2021;56:1221-33. [Crossref] [PubMed]
- Lopaschuk GD, Karwi QG, Tian R, et al. Cardiac Energy Metabolism in Heart Failure. Circ Res 2021;128:1487-513. [Crossref] [PubMed]
- Bertero E, Maack C. Metabolic remodelling in heart failure. Nat Rev Cardiol 2018;15:457-70. [Crossref] [PubMed]
- Horton JL, Martin OJ, Lai L, et al. Mitochondrial protein hyperacetylation in the failing heart. JCI Insight 2016;2:e84897. [Crossref] [PubMed]
- Lee CF, Chavez JD, Garcia-Menendez L, et al. Normalization of NAD+ Redox Balance as a Therapy for Heart Failure. Circulation 2016;134:883-94. [Crossref] [PubMed]
- Zhang X, Ji R, Liao X, et al. MicroRNA-195 Regulates Metabolism in Failing Myocardium Via Alterations in Sirtuin 3 Expression and Mitochondrial Protein Acetylation. Circulation 2018;137:2052-67. [Crossref] [PubMed]
- Yang H, Zhou L, Shi Q, et al. SIRT3-dependent GOT2 acetylation status affects the malate-aspartate NADH shuttle activity and pancreatic tumor growth. EMBO J 2015;34:1110-25. [Crossref] [PubMed]
- Yang Y, Wang W, Tian Y, et al. Sirtuin 3 and mitochondrial permeability transition pore (mPTP): A systematic review. Mitochondrion 2022;64:103-11. [Crossref] [PubMed]
- Hu Q, Zhang H, Gutiérrez Cortés N, et al. Increased Drp1 Acetylation by Lipid Overload Induces Cardiomyocyte Death and Heart Dysfunction. Circ Res 2020;126:456-70. [Crossref] [PubMed]
- Dey S, DeMazumder D, Sidor A, et al. Mitochondrial ROS Drive Sudden Cardiac Death and Chronic Proteome Remodeling in Heart Failure. Circ Res 2018;123:356-71. [Crossref] [PubMed]
- van der Pol A, van Gilst WH, Voors AA, et al. Treating oxidative stress in heart failure: past, present and future. Eur J Heart Fail 2019;21:425-35. [Crossref] [PubMed]
- Bayeva M, Gheorghiade M, Ardehali H. Mitochondria as a therapeutic target in heart failure. J Am Coll Cardiol 2013;61:599-610. [Crossref] [PubMed]
- Wong HS, Dighe PA, Mezera V, et al. Production of superoxide and hydrogen peroxide from specific mitochondrial sites under different bioenergetic conditions. J Biol Chem 2017;292:16804-9. [Crossref] [PubMed]
- Handy DE, Loscalzo J. The role of glutathione peroxidase-1 in health and disease. Free Radic Biol Med 2022;188:146-61. [Crossref] [PubMed]
- Quintana-Cabrera R, Manjarrés-Raza I, Vicente-Gutiérrez C, et al. Opa1 relies on cristae preservation and ATP synthase to curtail reactive oxygen species accumulation in mitochondria. Redox Biol 2021;41:101944. [Crossref] [PubMed]
- Li A, Zheng N, Ding X. Mitochondrial abnormalities: a hub in metabolic syndrome-related cardiac dysfunction caused by oxidative stress. Heart Fail Rev 2022;27:1387-94. [Crossref] [PubMed]
- Weissman D, Maack C. Redox signaling in heart failure and therapeutic implications. Free Radic Biol Med 2021;171:345-64. [Crossref] [PubMed]
- Marín-García J, Akhmedov AT, Moe GW. Mitochondria in heart failure: the emerging role of mitochondrial dynamics. Heart Fail Rev 2013;18:439-56. [Crossref] [PubMed]
- Bulua AC, Simon A, Maddipati R, et al. Mitochondrial reactive oxygen species promote production of proinflammatory cytokines and are elevated in TNFR1-associated periodic syndrome (TRAPS). J Exp Med 2011;208:519-33. [Crossref] [PubMed]
- Minutoli L, Puzzolo D, Rinaldi M, et al. ROS-Mediated NLRP3 Inflammasome Activation in Brain, Heart, Kidney, and Testis Ischemia/Reperfusion Injury. Oxid Med Cell Longev 2016;2016:2183026. [Crossref] [PubMed]
- Billingham LK, Stoolman JS, Vasan K, et al. Mitochondrial electron transport chain is necessary for NLRP3 inflammasome activation. Nat Immunol 2022;23:692-704. [Crossref] [PubMed]
- Huang Q, Zhou HJ, Zhang H, et al. Thioredoxin-2 inhibits mitochondrial reactive oxygen species generation and apoptosis stress kinase-1 activity to maintain cardiac function. Circulation 2015;131:1082-97. [Crossref] [PubMed]
- Hsieh CC, Li CY, Hsu CH, et al. Mitochondrial protection by simvastatin against angiotensin II-mediated heart failure. Br J Pharmacol 2019;176:3791-804. [Crossref] [PubMed]
- Rizzuto R, De Stefani D, Raffaello A, et al. Mitochondria as sensors and regulators of calcium signalling. Nat Rev Mol Cell Biol 2012;13:566-78. [Crossref] [PubMed]
- Singh S, Mabalirajan U. Mitochondrial calcium in command of juggling myriads of cellular functions. Mitochondrion 2021;57:108-18. [Crossref] [PubMed]
- Bertero E, Maack C. Calcium Signaling and Reactive Oxygen Species in Mitochondria. Circ Res 2018;122:1460-78. [Crossref] [PubMed]
- Gorski PA, Ceholski DK, Hajjar RJ. Altered myocardial calcium cycling and energetics in heart failure--a rational approach for disease treatment. Cell Metab 2015;21:183-94. [Crossref] [PubMed]
- Santulli G, Xie W, Reiken SR, et al. Mitochondrial calcium overload is a key determinant in heart failure. Proc Natl Acad Sci U S A 2015;112:11389-94. [Crossref] [PubMed]
- Zorov DB, Juhaszova M, Sollott SJ. Mitochondrial reactive oxygen species (ROS) and ROS-induced ROS release. Physiol Rev 2014;94:909-50. [Crossref] [PubMed]
- Kist M, Vucic D. Cell death pathways: intricate connections and disease implications. EMBO J 2021;40:e106700. [Crossref] [PubMed]
- Nakayama H, Chen X, Baines CP, et al. Ca2+- and mitochondrial-dependent cardiomyocyte necrosis as a primary mediator of heart failure. J Clin Invest 2007;117:2431-44. [Crossref] [PubMed]
- Morciano G, Naumova N, Koprowski P, et al. The mitochondrial permeability transition pore: an evolving concept critical for cell life and death. Biol Rev Camb Philos Soc 2021;96:2489-521. [Crossref] [PubMed]
- Bonora M, Patergnani S, Ramaccini D, et al. Physiopathology of the Permeability Transition Pore: Molecular Mechanisms in Human Pathology. Biomolecules 2020;10:998. [Crossref] [PubMed]
- Kwong JQ, Molkentin JD. Physiological and pathological roles of the mitochondrial permeability transition pore in the heart. Cell Metab 2015;21:206-14. [Crossref] [PubMed]
- Tanwar J, Singh JB, Motiani RK. Molecular machinery regulating mitochondrial calcium levels: The nuts and bolts of mitochondrial calcium dynamics. Mitochondrion 2021;57:9-22. [Crossref] [PubMed]
- Villalpando-Rodriguez GE, Gibson SB. Reactive Oxygen Species (ROS) Regulates Different Types of Cell Death by Acting as a Rheostat. Oxid Med Cell Longev 2021;2021:9912436. [Crossref] [PubMed]
- Chang TK, Shravage BV, Hayes SD, et al. Uba1 functions in Atg7- and Atg3-independent autophagy. Nat Cell Biol 2013;15:1067-78. [Crossref] [PubMed]
- Dasari SK, Bialik S, Levin-Zaidman S, et al. Signalome-wide RNAi screen identifies GBA1 as a positive mediator of autophagic cell death. Cell Death Differ 2017;24:1288-302. [Crossref] [PubMed]
- Weixler V, Lapusca R, Grangl G, et al. Autogenous mitochondria transplantation for treatment of right heart failure. J Thorac Cardiovasc Surg 2021;162:e111-21. [Crossref] [PubMed]
- Lutsenko S. Human copper homeostasis: a network of interconnected pathways. Curr Opin Chem Biol 2010;14:211-7. [Crossref] [PubMed]
- Ge EJ, Bush AI, Casini A, et al. Connecting copper and cancer: from transition metal signalling to metalloplasia. Nat Rev Cancer 2022;22:102-13. [Crossref] [PubMed]
- Chen J, Jiang Y, Shi H, et al. The molecular mechanisms of copper metabolism and its roles in human diseases. Pflugers Arch 2020;472:1415-29. [Crossref] [PubMed]
- Cvetinovic N, Loncar G, Isakovic AM, et al. Micronutrient Depletion in Heart Failure: Common, Clinically Relevant and Treatable. Int J Mol Sci 2019;20:5627. [Crossref] [PubMed]
- McKeag NA, McKinley MC, Woodside JV, et al. The role of micronutrients in heart failure. J Acad Nutr Diet 2012;112:870-86. [Crossref] [PubMed]
- Koşar F, Sahin I, Taşkapan C, et al. Trace element status (Se, Zn, Cu) in heart failure. Anadolu Kardiyol Derg 2006;6:216-20. [PubMed]
- Shokrzadeh M, Ghaemian A, Salehifar E, et al. Serum zinc and copper levels in ischemic cardiomyopathy. Biol Trace Elem Res 2009;127:116-23. [Crossref] [PubMed]
- Ghaemian A, Salehifar E, Jalalian R, et al. Zinc and copper levels in severe heart failure and the effects of atrial fibrillation on the zinc and copper status. Biol Trace Elem Res 2011;143:1239-46. [Crossref] [PubMed]
- Málek F, Dvorák J, Jiresová E, et al. Difference of baseline serum copper levels between groups of patients with different one year mortality and morbidity and chronic heart failure. Cent Eur J Public Health 2003;11:198-201. [PubMed]
- Chrysochou E, Kanellopoulos PG, Koukoulakis KG, et al. Heart Failure and PAHs, OHPAHs, and Trace Elements Levels in Human Serum: Results from a Preliminary Pilot Study in Greek Population and the Possible Impact of Air Pollution. Molecules 2021;26:3207. [Crossref] [PubMed]
- Kunutsor SK, Voutilainen A, Kurl S, et al. Serum copper-to-zinc ratio is associated with heart failure and improves risk prediction in middle-aged and older Caucasian men: A prospective study. Nutr Metab Cardiovasc Dis 2022;32:1924-35. [Crossref] [PubMed]
- de Lorgeril M, Salen P, Accominotti M, et al. Dietary and blood antioxidants in patients with chronic heart failure. Insights into the potential importance of selenium in heart failure. Eur J Heart Fail 2001;3:661-9. [Crossref] [PubMed]
- Bomer N, Grote Beverborg N, Hoes MF, et al. Selenium and outcome in heart failure. Eur J Heart Fail 2020;22:1415-23. [Crossref] [PubMed]
- Mirdamadi A, Rafiei R, Kahazaipour G, et al. Selenium Level in Patients with Heart Failure versus Normal Individuals. Int J Prev Med 2019;10:210. [Crossref] [PubMed]
- Zhang Z, Chang C, Zhang Y, et al. The association between serum selenium concentration and prognosis in patients with heart failure in a Chinese population. Sci Rep 2021;11:14533. [Crossref] [PubMed]
- Al-Mubarak AA, Grote Beverborg N, Suthahar N, et al. High selenium levels associate with reduced risk of mortality and new-onset heart failure: data from PREVEND. Eur J Heart Fail 2022;24:299-307. [Crossref] [PubMed]
- Cooper GJ. Selective divalent copper chelation for the treatment of diabetes mellitus. Curr Med Chem 2012;19:2828-60. [Crossref] [PubMed]
- Belyaeva EA, Sokolova TV, Emelyanova LV, et al. Mitochondrial electron transport chain in heavy metal-induced neurotoxicity: effects of cadmium, mercury, and copper. ScientificWorldJournal 2012;2012:136063. [Crossref] [PubMed]
- Borchard S, Bork F, Rieder T, et al. The exceptional sensitivity of brain mitochondria to copper. Toxicol In Vitro 2018;51:11-22. [Crossref] [PubMed]
- Cooper GJ, Phillips AR, Choong SY, et al. Regeneration of the heart in diabetes by selective copper chelation. Diabetes 2004;53:2501-8. [Crossref] [PubMed]
- Rowland EA, Snowden CK, Cristea IM. Protein lipoylation: an evolutionarily conserved metabolic regulator of health and disease. Curr Opin Chem Biol 2018;42:76-85. [Crossref] [PubMed]
- Neagle J, De Marcucci O, Dunbar B, et al. Component X of mammalian pyruvate dehydrogenase complex: structural and functional relationship to the lipoate acetyltransferase (E2) component. FEBS Lett 1989;253:11-5. [Crossref] [PubMed]
- Cui L, Gouw AM, LaGory EL, et al. Mitochondrial copper depletion suppresses triple-negative breast cancer in mice. Nat Biotechnol 2021;39:357-67. [Crossref] [PubMed]
- Brady DC, Crowe MS, Greenberg DN, et al. Copper Chelation Inhibits BRAFV600E-Driven Melanomagenesis and Counters Resistance to BRAFV600E and MEK1/2 Inhibitors. Cancer Res 2017;77:6240-52. [Crossref] [PubMed]
- Wang T, Guo Z. Copper in medicine: homeostasis, chelation therapy and antitumor drug design. Curr Med Chem 2006;13:525-37. [Crossref] [PubMed]
- Zhang Z, Ma Y, Guo X, et al. FDX1 can Impact the Prognosis and Mediate the Metabolism of Lung Adenocarcinoma. Front Pharmacol 2021;12:749134. [Crossref] [PubMed]
- Tsvetkov P, Detappe A, Cai K, et al. Mitochondrial metabolism promotes adaptation to proteotoxic stress. Nat Chem Biol 2019;15:681-9. [Crossref] [PubMed]
- Cai K, Tonelli M, Frederick RO, et al. Human Mitochondrial Ferredoxin 1 (FDX1) and Ferredoxin 2 (FDX2) Both Bind Cysteine Desulfurase and Donate Electrons for Iron-Sulfur Cluster Biosynthesis. Biochemistry 2017;56:487-99. [Crossref] [PubMed]
- Bian Z, Fan R, Xie L. A Novel Cuproptosis-Related Prognostic Gene Signature and Validation of Differential Expression in Clear Cell Renal Cell Carcinoma. Genes (Basel) 2022;13:851. [Crossref] [PubMed]
- Roger VL. Epidemiology of Heart Failure: A Contemporary Perspective. Circ Res 2021;128:1421-34. [Crossref] [PubMed]
- Rosca MG, Hoppel CL. Mitochondria in heart failure. Cardiovasc Res 2010;88:40-50. [Crossref] [PubMed]
- Marín-García J, Akhmedov AT. Mitochondrial dynamics and cell death in heart failure. Heart Fail Rev 2016;21:123-36. [Crossref] [PubMed]
- Marín-García J, Goldenthal MJ. Mitochondrial centrality in heart failure. Heart Fail Rev 2008;13:137-50. [Crossref] [PubMed]