ATF3 and its emerging role in atherosclerosis: a narrative review
Introduction
Cardiovascular disease, such as ischemic heart disease and stroke, is the most common single cause of death worldwide, and its morbidity and mortality rates continue to increase (1). Atherosclerosis (AS) is one of the main types of arteriosclerotic vascular disease, and carries a considerable risk of ischemic diseases including ischemic stroke, myocardial infarction and disabling peripheral artery disease (2). Characteristically in AS, the barrier of endothelium becomes impaired, resulting in subintima lipid accumulation, macrophage infiltration, and the proliferation of vascular smooth muscle cells (VSMCs), which subsequently cause foam cell transformation and plaque formation (3). The major risk factors of AS regulator of the cellular include being overweight, physical inactivity, type 2 diabetes mellitus (T2DM), hyperlipidemia, tobacco use and hypertension (4). Traditionally, AS was defined as chronic vascular inflammation caused by the abnormal retention of lipoproteins, especially the low-density lipoprotein (LDL) cholesterol. In recent years, research has shown that the development of AS comprises a series of intricate pathological processes, that are affected by both vascular intrinsic factors and many other risk factors extrinsic to the vasculature (5). Additionally, multiple lines of evidence have shown that transcriptional factors, which play a critical role in vascular homeostasis and metabolic regulation, represent promising therapeutic targets for the prevention and treatment of AS (6).
As a pivotal transcriptional factor belonging to the activating transcription factor (ATF)/cyclic adenosine monophosphate response element binding protein (CREB) family family, ATF3 is considered a master in glioma cells adaptive-response network (7,8). The function and expression of ATF3 is governed by transcriptional regulation, post transcriptional control and post-translational modification (PTM). In the context of the stress response, ATF3 is stimulated and produces transcriptional inhibitory or stimulatory effects by forming homodimers or heterodimers with other ATF/CREB members (9,10). Under physiological conditions, ATF3 expression is always transient but crucial to cell-cycle regulation and metabolic homeostasis (11). The sustained overexpression or deficiency of ATF3 may contribute to the occurrence of disease. For example, ATF3 deletion has been shown to exacerbate ischemia/reperfusion injury in the liver due to its promotion of mammalian target of rapamycin (mTOR) and phosphoprotein 70 ribosomal protein S6 kinase (p70S6K) via toll-like receptor 4 (TLR4)-driven inflammatory responses and T cell development (12). However, the overexpression of ATF3 has also been found to participate in particulate matter-induced airway inflammation in mouse lung tissues by promoting inflammatory cytokine expression such as interleukin (IL)-6 through the nuclear factor kappa B (NF-κB) and activator protein 1 (AP-1) pathways. Additionally, the alleviation of neutrophil infiltration and decreased expression of IL-6 and CXCL2 has been observed in the lung of ATF3-/- mice (13). Interestingly, the functional complexity of ATF3 has also been observed in various pathological stages of AS. It has been well established that ATF3 is associated with the pathogenesis of AS, however, the specific effect of ATF3 activation in AS remains controversial. Qin et al. conducted a bioinformatics analysis and identified ATF3 as a novel biomarker for the prognosis of AS. Compared with the ruptured atherosclerotic plaques, lower expression levels of ATF3 were found in macrophages from stable atherosclerotic plaques, which indicates ATF3 expression in macrophages is correlated with the stability of atherosclerotic plaques (14). Further, the induction of CREB/ATF3 signaling via uremic toxin dramatically aggravates VSMC proliferation and neointimal formation (15). The expression of ATF3 was also observed to be increased in the atherosclerotic plaques of uremic apolipoprotein E (ApoE)-/- mice and iliac arteries of uremic patients (15). There is extensive evidence that ATF3 also participates in atherogenesis by affecting vascular homeostasis when hepatocyte-specific ATF3 overexpression significantly reduces the development of AS through its improvement of lipid metabolism (16,17). Given the crucial role of ATF3 in the pathology of AS, it is of great significance to examine the precise impact of ATF3 dysfunction in various stages of AS.
Although further research which aims to elucidate the role of ATF3 in vascular pathology and metabolic regulation remains to be conducted, research on its involvement in atherogenesis, plaque progression, and plaque stability has recently led to a series of breakthroughs. Given the importance and complexity of the ATF3-related mechanism in AS, we sought to summarize the current advances in the specific mechanism of ATF3 activation and the main contribution to ATF3 in AS, highlighting the vascular intrinsic and extrinsic mechanisms of how ATF3 affects the pathology of AS. We present the following article in accordance with the Narrative Review reporting checklist (available at https://cdt.amegroups.com/article/view/10.21037/cdt-22-206/rc).
Methods
In this paper, the literature retrieval was performed in the PubMed database to search the English publication correlated to the regulatory mechanism of ATF3 and the specific role of ATF3 in AS from origin to March 2022. The major of search terms and search strategy summary are shown in Table 1 and Table 2, respectively.
Table 1
“Activating Transcription Factor 3”[MeSH] |
(“Activating Transcription Factor 3”[MeSH]) AND (“Alternative Splicing”[MeSH]) |
(“Activating Transcription Factor 3”[MeSH]) AND (“transcription”[MeSH]) |
(“Activating Transcription Factor 3”[MeSH]) AND (“RNA Processing, Post-Transcriptional”[MeSH]) |
(“Activating Transcription Factor 3”[MeSH]) AND (“Protein Processing, Post-Translational”[MeSH]) |
(“Activating Transcription Factor 3”[MeSH]) AND (“Atherosclerosis”[MeSH]) |
(“Activating Transcription Factor 3”[MeSH]) AND (“Endothelial Cells”[MeSH]) |
(“Activating Transcription Factor 3”[MeSH]) AND (“Macrophage”[MeSH]) |
(“Activating Transcription Factor 3”[MeSH]) AND (“Vascular Remodeling”[MeSH]) |
(“Activating Transcription Factor 3”[MeSH]) AND (“Foam Cells”[MeSH]) |
(“Activating Transcription Factor 3”[MeSH]) AND (“Plaque, Atherosclerotic”[MeSH]) |
(“Activating Transcription Factor 3”[MeSH]) AND (“Hyperglycemia”[MeSH]) |
(“Activating Transcription Factor 3”[MeSH]) AND (“Dyslipidemias”[MeSH]) |
(“Activating Transcription Factor 3”[MeSH]) AND (“Immunity”[MeSH]) |
Table 2
Item | Specification |
---|---|
Date of search | 2022/02/11–2022/03/07 |
Databases and other sources searched | PubMed |
Search terms used | As mentioned in Table 1 |
Timeframe | 1994–2022 |
Inclusion and exclusion criteria | Inclusion criteria: original article and reviews in English about the regulatory mechanism of ATF3 and the specific role of ATF3 in AS |
Exclusion criteria: article or review which are not related with ATF3 nor AS | |
Selection process | Selection was conducted independently by Huang Yifan and Wu Jingyi. the data of search were discussed with other authors and checked by Yang Jian |
AS, atherosclerosis.
Basic structure and function of ATF3
As a member of the ATF/CREB family of transcription factors, ATF3 consists of 181 amino acid residues with a molecular mass of 22 kD (18). When the heterodimers of ATF3 form with other ATF/CREB members and show the effects of transcriptional inhibition or activation, ATF3 acts as a transcriptional repressor by forming a homodimer, depending on the cell type and promoter. The known binding sequences of ATF3 on the target promoters include the cyclic AMP response element (TGACGTCA), IL-12p40 (TCGACGTCT), cyclin D1 (TAACGTCA), Growth Arrest And DNA Damage-Inducible Protein GADD153 (TTGCATCA) and macrophage inflammatory protein-1 beta (TGACATCA) (19-23). Further, ATF3 can also modulates cell function by interacting with other proteins independently of its transcriptional activity. For example, ATF3 directly binds to the C-terminus of protein 53 (P53) directly and prevent it from murine double minute 2 (MDM2)-mediated ubiquitination through competitive inhibition, thereby acting as a tumor suppressor of DNA damage (24).
Multiple transcript variants of ATF3 have been reported to participate in the regulation of cell function, including mitochondrial oxidative stress-induced transcript variants (ATF3-T4 and ATF3-T5) and alternative splicing variants such as ATF3Δzip, ATF3Δzip2 (ATF3Δzip2a and ATF3Δzip2b), ATF3Δzip2c, ATF3Δzip3, and ATF3b (Figure 1) (25,26). Both ATF3-T4 and ATF3-T5 have been identified as key contributors of endothelial inflammation and lipotoxic brain microvascular injury (25). ATF3Δzip2 is a promoter of apoptotic cell death, and has recently been found to be involved in rat skeletal muscle energy metabolism (27,28). ATF3deltaZip2c and ATF3deltaZip3 are stimulated by amino acid deprivation and may have a potential role in nutrient metabolism of human hepatocellular carcinomas (HepG2) cells, And ATF3b has been identified in the alpha cells of the pancreatic islets, which are involved in glucose modulation by positively regulating the synthesis and secretion of glucagon (29,30).
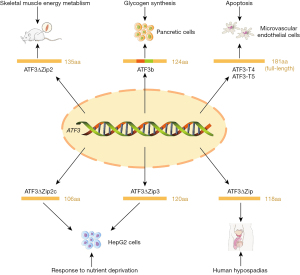
In the maintenance of homeostasis, ATF3 functions as a hub of the cellular adaptive-response network, which plays an important role in inflammation, immune response, oncogenesis, and nutrient metabolism (26). The abnormalities of ATF3 expression and regulation have been observed in the pathogenesis of various diseases, such as cancer, cardiac remodeling, rheumatoid arthritis, and microvascular injury (25,31-33). Recent studies have shown that the upregulation and nuclear translocation of ATF3 promote ferroptosis, a form of regulated cell death characterized by iron-dependent lipid peroxidation. Lu et al. found that the overexpression of ATF3 and subsequent hydrogen peroxide (H2O2) accumulation contributes to brucine-induced ferroptosis in glioma cells (34). Additionally, Wang et al. confirmed that treatment with erastin in vitro induces ATF3 expression and depletion of the amino acid antiporter system Xc-, thus resulting in a decrease in the intracellular glutathione (GSH) level and triggers cell ferroptosis. The suppression of Xc- by ATF3 is partly due to its transcriptional repression for solute carrier family 7 member 11 (SLC7A11) (35). These findings indicate that ATF3 plays a central role in oxidative stress and ferroptosis, and suggests a potential link between ATF3 and ferroptosis-related disease, such as AS.
The specific mechanism for ATF3 regulation
As mentioned above, ATF3 performs various, or even opposite functions in different cells, which may be related to its activation pathway. Previous research has revealed that stress signals, such as hypoxia, cytokines, cAMP, calcium influx, ultraviolet (UV) irradiation, and the unfolded protein response, play an important role in the induction of ATF3 (36-38). As research on ATF3 continues, increasing evidence has indicated that the activity and function of ATF3 are regulated by a complex network involving transcriptional regulation, post-transcriptional control, and PTM (Figure 2).
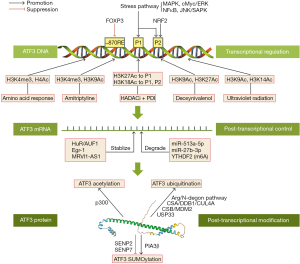
Transcriptional regulation
The transcriptional activity of ATF3 is mainly controlled by its promoters, the specific binding sequence of RNA polymerase. A study by Miyazaki et al. revealed that 2 promoters control transcriptional activity within the human ATF3 gene. Apart from the canonical promoter P2, they described an alternate promoter P1 localized about 43.5-kb upstream of the P2 promoter, which is evolutionally conserved and has a significantly different structure to the P2 promoter, and comparative differences in the intensity of activation upon various stimuli (39). Additionally, extensive research has shown that stress-related pathways including the mitogen-activated protein kinase (MAPK), NF-κB, Myc proto-oncogene protein (cMyc)/extracellular signal-related kinase (ERK), and Jun N-terminal kinase (JNK)/stress- and mitogen-activated protein kinase (SAPK) play a crucial role in ATF3 activation through the direct binding of their downstream effectors to ATF promoter (40-43). Interestingly, in recent years, some transcription factors closely related to the pathogenesis of AS have also been reported to be involved in the transcriptional regulation of ATF3. Forkhead Box Protein P3 (FOXP3), which is a crucial contributor to the atheroprotective regulatory T cell (Treg) phenotype, represses the ATF3 transcriptional activity by directly binding to the −870 response element (RE) region on the ATF3 promoter (44,45). Nuclear factor E2-related factor 2 (Nrf2), which modulates both early atherogenesis and macrophage phenotype in late advanced atherosclerotic lesion, was also found to induce the transcriptional activation of both full length ATF3 and splicing variant ATF3ΔZip2 (46). Additionally, chromatin immunoprecipitation (ChIP) assays have revealed that activating NRF2 leads to the induction of the ATF3 proximal promoter (P2 promoter described above), which mainly results in the up-regulation of ATF3ΔZip2 (47).
In addition to the transcriptional factors, the transcription of ATF3 is also regulated by epigenetic events. The levels of histone 3 trimethylated Lys4 and histone acetylated histone 4 were observed to be increased in the AFT3 gene during the amino acid response, and a concurrent loss of total H3 protein near the promoter was also observed (48). Further, Tran et al. found that pre-treatment with amitriptyline caused anti-apoptotic effects in mouse neuronal cells by positively regulating the acetylation level of histone H3 lysine 9 and trimethylation of histone H3 lysine 4 in the promoter regions of ATF3 (49). It is likely that histone deacetylase inhibitors (HDACis) can be used as target agents for several cancers through the induction of ATF3 and pro-apoptosis, and these findings suggest a potential association between ATF3 activation and histone acetylation (50). Duncan et al. discovered that the combination of HDACis and protein disulfide isomerase inhibitors (PDIs) produced a 113-fold induction of ATF3 through increased histone acetylation and RNA polymerase II recruitment. The ChIP assays further confirmed that the acetylated histone 3 lysine 27 (H3K27-ac) and histone 3 lysine 18 (H3K18-ac) marks were significantly enriched in the upstream regulatory regions that flank the ATF3 transcription start site in human pancreatic adenocarcinoma cells (PANC-1) treated with the combination of HDACi and PDI (51). Additionally, the striking enrichment of H3K27-ac was observed at distal promoter P1 of ATF3, while RNA polymerase II was recruited at proximal promoter P2 and H3K18-ac surrounded both promoter regions (51). However, the deoxynivalenol-induced H3K9-ac and H3K27-ac enrichment, which gave rise to the upregulation of ATF3ΔZip2a/2 and subsequent G2/M cell cycle arrest in the HepG2 cells, did not differ significantly between the P1 and P2 promoters of ATF3 (52). Further, exposure to UV radiation activates ATF3 transcription by increasing the histone H3K9⁄K14-ac levels in promoter regions, the effect of which was entirely inhibited by 100µM of curcumin treatment (53). Further evidence also links DNA methylation to ATF3 transcriptional regulation, the hypermethylation of ATF3, and the subsequent down-regulation of ATF3 and the expression of ADP-ribosylation factor like-4 has been observed in tumor samples from recent research (54).
Post-transcriptional control
Current studies have indicated that the modulation of ATF3 in post-transcriptional control involves the RNA-binding protein, microRNA, RNA methylation, and long non-coding RNA (lncRNA). RNA-binding protein human antigen R enhances the stability of ATF3 messenger RNA (mRNA) in conjunction with AU-rich element RNA binding protein 1 (AUF1), which contributes to increases in expression following amino acid limitation (55). While the binding site remains unclear, He et al. demonstrated that early growth response gene 1 (EGR-1) mediates ATF3 overexpression by stabilizing ATF3 mRNA in glomerular mesangial cells (GMCs) upon sublytic C5b-9 stimulation (56). Moreover, recent studies have shown the pivotal role of the lncRNA-miRNA-mRNA network in ATF3 regulation. A weighted gene co-expression network analysis identified the nuclear paraspeckle assembly transcript 1 (NEAT1)/X inactive specific transcript (XIST)/potassium voltage-gated channel subfamily Q member 1 (KCNQ1T1)-miR-27a-3p/miR-16-5p-ATF3 axis as a potential RNA regulatory pathway in early diabetic nephropathy (57). Zhu et al. confirmed that the overexpression of lncRNA murine retrovirus integration site 1 homolog antisense RNA 1 (MRVI1-AS1) increases nasopharyngeal carcinoma (NPC) paclitaxel chemosensitivity by enhancing ATF3 expression via the suppression of miR-27b-3p and miRNA-513a-5p (58). Further, the genome-wide profiling of N6-methyladenosine (m6A)-tagged mRNA in adult dorsal root ganglion showed that the m6A-based regulation contributes to ATF3 activation and subsequent axon regeneration in adult mammal samples (59). Liu et al. found a novel mechanism for the disruption of ATF3 mRNA stabilization through m6A methylation. They identified m6A reader protein YTHDF2 as the key regulator of m6A modification and demonstrated that decreased m6A methylation at the A131 site in the 5'-untranslated region of ATF3 mRNA and the ensuing ATF3 overexpression mediates the tamoxifen resistance of human breast cancer cells (MCF-7) via the overexpression of ATP binding cassette subfamily B member 1 (ABCB1) (60).
PTM
As mentioned above, the activity of ATF3 protein mainly depends on the PTMs, including acetylation, SUMOylation, and ubiquitination. The histone acetyltransferase E1A binding protein p300 (P300) was observed to acetylate ATF3 at K42, thereby inducing the apoptosis of GMCs in Thy-1N rats treated with sublytic C5b-9 (56). Similarly, the SUMOylation of ATF3 at K42, which is enhanced by SUMO E3 ligase protein inhibitor of activated STAT 3β (PIAS3β) but decreased by SUMOylation protease SUMO specific peptidase 2 (SENP2) and SUMO specific peptidase 7 (SENP7), promote ATF3 activity by increasing protein stability (61). Later work on ATF3 SUMOylation suggests that the endogenous SUMO modification of ATF3 is implicated in the pathogenesis of hypertension and prostate cancer (62,63). Conversely, ATF3 ubiquitination mediates the degradation of the ATF3 protein, which was previously thought to be strongly correlated with a reduced expression and loss of function. However, a recent study by Li et al. interestingly speculated that ATF3 may stabilize the p53 protein by directly binding to the MDM2 RING domain and competing with p53 for MDM2-mediated ubiquitination (24). Additionally, ChIP assays have shown that the ubiquitin degradation of ATF3 is regulated by both the Cockayne syndrome protein A (CSA)/damage specific DNA binding protein 1 (DDB1)/Cullin 4A (CUL4A) ubiquitin ligase complex and the cockayne syndrome B protein (CSB)/MDM2 containing complex together (64). Other newly discovered mechanisms of ATF3 ubiquitination and stabilization comprise the ATF3 conditional degradation by the Abelson-related gene protein /N-degron pathway and ubiquitin-specific peptidase 33-mediated ATF3 deubiquitination (65,66).
In summary, it can be concluded that the transcript variants of ATF3 and different response of the 2 promoters upon various stimuli mainly contributes to the regulatory diversity of ATF3. However, only a few studies have focused on differential regulation in post-transcriptional control and PTM for different ATF3 transcript variants. Further research is required to investigate the specific mechanism regulating the mRNA stabilization and protein modification of ATF3 transcript variants. Consideration also needs to be given to the crosstalk and interaction among the multiple pathways of ATF3 ubiquitination and degradation, which may create a new field for the pharmacological intervention of ATF3 in the presence of disease.
The function and molecular mechanism of ATF3 in AS
Vascular intrinsic mechanisms of ATF3 in AS
The main characteristic features of AS are atherosclerotic plaques on the inside wall of the arteries and narrowed arterial lumen. During the progression of AS, the arterial lesions are mainly affected by the functional status of the endothelial cells (ECs), macrophages and VSMCs, whose abnormality leads to vascular endothelial disorder and contributes to plaque formation (2). ATF3 is a transcription factor that show differential expression in atherosclerotic plaque tissue. The existing evidence supports the notion that ATF3 is involved in the pathology of atherosclerotic vasculopathy by regulating endothelial dysfunction, macrophage polarization, foam cell formation, and vascular remodeling (Figure 3).
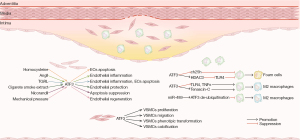
Endothelial dysfunction
Endothelial dysfunction occurs in the early stage of AS, in which ATF3 plays an extremely complicated role. A comparative study of immune-histological assay found that ATF3 was significantly overexpressed in the vascular ECs of human atherosclerotic lesions (67). Aung et al. found that lipolysis products of triglyceride-rich lipoproteins (TRLs) induce vascular inflammation and EC apoptosis via the nuclear expression of phosphorylated JNK and ATF3 (68). The lipolysis products induce caspase-3/7 activity and the induction of E-selectin, IL-8, IL-1α, Kruppel-like factor 4 (KLF4), and vascular endothelial growth factor (VEGF) were significantly suppressed after ATF3 blockade in vitro while the resistance of vascular apoptosis was shown in ATF3(-/-) mice treated with TGRL lipolysis (68). Homocysteine, an independent risk factor of AS, was found to activate endoplasmic reticulum stress and upregulate ATF3 expression via IRE1/TRAF2 pathway, thereby inducing apoptosis in human umbilical vein ECs (HUVECs) (69). In another study, Zhang et al. revealed the pivotal role of ATF3 SUMOylation in angiotensin II (Ang II)-induced endothelial injury. Ang II-induced ATF3 SUMOylation exacerbates endothelial inflammation by inhibiting endothelial nitric oxide (NO) synthase and NO production (62). Additionally, the vasodilatation of the aorta was improved in Ang II-induced hypertensive mice after treatment with a SUMOylation inhibitor (62). These observations suggest that ATF3 acts as a contributor to endothelial dysfunction and AS by promoting effect in EC inflammation and apoptosis.
Nonetheless, there is mounting evidence that ATF3 physiologically provides endothelial protection. Teasdale et al. described an ATF3-mediated protective effect in attenuating inflammation through an NF-κB independent pathway in ECs treated with cigarette smoke extract (70). McDonald et al. also showed the importance of ATF3 activation in endothelial proliferation and regeneration through an in vivo aortic endothelial injury model (71). A comparative study of transcriptional profile between ATF3 positive and negative cells suggested that ATF3 regulates endothelial regeneration through a cohort of early stress response genes including Fox, Jun, Egr1, Klf4, and Klf2 (71). Furthermore, ATF3 has been shown to play an essential role in nicorandil-induced endothelial protection. Silencing ATF3 with small-interfering RNA was shown to significantly impair the inhibitory effects of nicorandil on doxorubicin-induced endothelial apoptosis through Nrf2/Heme Oxygenase 1 signaling pathway (72).
In relation to the dual role of ATF3 in vascular endothelial homeostasis, the study by Nyunt et al. revealed a probable hypothesis that the selective expression of ATF3 transcript variants under different conditions is responsible for the diverse effects of ATF3 in the endothelium. They found that 2 ATF3 transcript variants (i.e., ATF3-T4 and ATF3-T5) are which were selectively up-regulated in response to TGRL lipolysis products and drive EC apoptosis through the mitochondrial pathway (25). However, whether other ATF3 transcript variants are involved in the endothelial regulation remains unknown. Additional studies are demanded in the specific activation mechanisms and functional characteristics of each ATF3 transcript variant in vascular endothelium.
Macrophage polarization
The polarization and corresponding phenotype of migrated macrophages in atherosclerotic plaques significantly affect the size and stability of plaques through the entire process of AS (73). The transgenic overexpression of ATF3 in macrophages effectively attenuate obesity-induced induced M1 polarization in obese adipose tissue (74). A ChIP analysis revealed that ATF3 was recruited to the promoter region of tumor necrosis factor-α (TNF-α) by saturated fatty acids, thus resulting in the transcriptional repression of saturated fatty acids/TLR4 signaling and TNF-α (74). Sha et al. further confirmed that ATF3 overexpression promotes macrophage migration and transforms M1‑polarized macrophages to the M2 phenotype by activating tenascin‑C, which is significantly suppressed by the inhibition of Wnt/βcatenin signaling (75). It has been reported that M2 macrophages play a preventive role in the progression of AS, the ATF3 activation appears to exert macrophage polarization into an anti-inflammatory phenotype in AS. However, Li et al. recently proposed that the reduced M2-like polarization of macrophages mediated by miR-489 is associated with ATF3 deubiquitination through MDM2 (76). These findings suggest that ATF3 has a complex role in macrophage polarization. A Kyoto Encyclopedia of Genes and Genomes (KEGG) analysis revealed a positive correlation between expression levels of ATF3 in macrophage and the stability of atherosclerotic plaques (14).
Foam cell formation
Foam cell formation and accumulation in the artery wall are critical events in atherosclerotic plaque development. Research has shown that ATF3 activation controls the macrophage-derived foam cell formation by repressing Ch25h through increased histone acetylation at its promoter (77). An increased severity of AS in the aortic root and greater neutral lipid content in peritoneal macrophage have been observed in Atf3−/−Apoe−/− mice fed a high-fat diet (HFD) for 8 weeks (77). Luo et al. revealed that the inhibitory effect of ATF3 on lipopolysaccharide-induced macrophage inflammation and foam cell formation is mainly the result of TLR-4 suppression (78). Additionally, another study suggested that ATF3 may diminish the TLR4-mediated hyperinflammatory response to LPS in macrophages by up-regulating HDAC3-mediated histone deacetylation (79). Another major source of foam cells in AS is VSMC. The specific effect of ATF3 in VSMC-derived foam cells has not yet been well established, however, the latest evidence supports the notion that ATF3 functioned as a key repressor for the transformation of VSMCs into atherogenic phenotype in atherosclerotic lesion (16). Phenome-wide association studies also indicated that the reduced expression of ATF3 is correlated with an increased risk for AS (16). More research is now needed to confirm and explore the link between ATF3 expression and VSMC-derived foam cells.
Vascular remodeling
Pathological vascular remodeling is a hallmark of AS. Meanwhile, the vascular remodeling, especially in hypertension, has also been considered a key cause of atherosclerotic lesions. Studies have found that the upregulation of ATF3 leads to neointima formation and vascular calcification by promoting the proliferation and calcium deposition of VSMCs (15,80). In a model of hypertension-induced vascular remodeling, ATF3 was observed to partly mediate mechanical stretch-induced VSMC proliferation, migration, apoptosis, and phenotypic transformation through the transcriptional suppression of ACE2, a protective factor widely expressed in the human aorta (81). Such findings indicate that ATF3 is a key promoter of cardiovascular remodeling, and is correlated with a higher risk of atherogenesis and plaque formation. However, the study by Wang et al. has shown that ATF3 is inversely associated with atherosclerotic progression through genome-wide association analysis, which suggests that the effect of ATF3 in VSMCs is complex and dynamic (16).
Vascular extrinsic mechanisms of ATF3 in AS
The understanding of AS has evolved substantially in the last few decades. Emerging evidence highlights the vital role of vascular extrinsic factors in AS. In addition to vascular homeostasis, ATF3 also affects atherogenesis and AS progression via its effects on hyperglycemia, dyslipidemia, and the immune response (Figure 4).
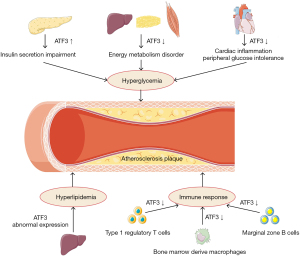
Hyperglycemia
Hypoglycemic therapy has proven to be ineffective in limiting vascular complications that result from T2DM, however, hyperglycemia is still regarded as a crucial contributor to DM-induced AS (82). Some studies have noted the discrepant roles of ATF3 in glucose metabolism, which may be attributed to the varied effects of ATF3 expression in various organs and tissues. In the pancreatic β-cells of both diabetic humans and mice with developed insulitis or DM, stress-induced ATF3 expression stimulates cell apoptosis and impairs insulin secretion (83). In vitro research has also confirmed that the knockdown of ATF3 safeguards islets and glucose homeostasis in a mouse transplantation model, which suggests that ATF3 has a potential role in islet injury and subsequent disorder of glucose metabolism (84). Some studies have found that transcription factor JunB-induced ATF3 upregulation protects against pancreatic β-cells apoptosis triggered by pro-inflammatory cytokines TNF-a and interferon-gamma, in vitro research has shown that both glucose sensitivity and insulin secretion in rat pancreatic β-cells is unaffected by ATF3 knockout (KO) (85,86). However, ATF3 KO mice have displayed impaired glucose tolerance and morbid obesity when fed HFD, which is accompanied by an increased risk of AS (87). The conflicting results could be partly explained by the crucial role of ATF3 in energy homeostasis. Notably, ATF3 expression has been found to be more reduced in the liver, adipose tissue, and muscle specimens of obese patients than normal patients (88). Further, both genetic and pharmacologic upregulation of ATF3 in adipocytes ameliorates HFD-induced insulin resistance and glucose intolerance by promoting adipocyte browning (88). The overexpression of ATF3 in 3T3-Ll cells was shown to reduce the expression of markers related to adipogenesis and lipogenesis, including peroxisome proliferator activated receptor gamma (PPARγ), CCAAT/enhancer-binding protein alpha (c/EBPα), acetyl-CoA carboxylase 1/2 (ACC1/2), carbohydrate response element binding protein (ChREBP) and stearoyl-coA desaturase 1 (SCD1), while the BAT/beige and β-oxidation markers, such as uncoupling protein 1 (UCP1), PPAR gamma coactivator 1 alpha (PGC1α), carnitine palmitoyltransferase 1a (Cpt1α), and medium-chain Acyl-CoA dehydrogenase (Mcad) were found to be markedly upregulated (89). Further, cardiac-specific ATF3 deficiency exacerbates peripheral glucose intolerance by increasing cardiac inflammation, which triggers the secretion of cardiac inflammatory cytokines IL-6 and TNFα and impaired insulin signaling (89). Nevertheless, hepatic ATF3 expression was shown to seriously undermine glucose homeostasis in both an acute alcoholic liver injury model and a non-alcoholic fatty liver disease model, indicating the adverse effect of ATF3 on hepatic energy metabolism (90,91). In Zucker diabetic fatty (ZDF) rats, ATF3 was shown to induce insulin resistance and mitochondrial dysfunction with decreased fatty acid oxidation rates, increased mitochondrial reactive oxygen species (ROS) production, and adenosine triphosphate (ATP) depletion while ATF3 directly injured hepatic glucose production by down-regulating the expression of gluconeogenic genes such as glucose-6-phosphatase catalytic (G6pc), phosphoenolpyruvate carboxykinase 1 (Pck1), and Pgc1α (90.91). Lee et al. also observed higher insulin sensitivity in pancreas- and hypothalamus-specific ATF3 KO (PHT-ATF3-KO) mice, which was associated with decreased food intake and increased energy expenditure rather than plasma glucagon or insulin (92).
Dyslipidemia
The vital role of dyslipidemia in the initiation and progression of AS has been well documented. The deposition of lipid particles in the arterial wall and lipids-induced foam cell formation are considered critical events of atherogenesis and plaque progression, and may be exacerbated under the condition of dyslipidemia (93). It has been revealed that ATF3 deficiency is closely associated with metabolic syndrome and vascular homeostasis (94). Compared to wild-type mice, higher serum levels of triglycerides, glucose, insulin, and inflammatory cytokines were observed in ATF3-KO mice fed with fructose, as well as more intense increases in aorta wall remodeling and lipid peroxide levels (94). Although recent studies have established the potential role of hepatic ATF3 expression in dyslipidemia, controversy lingers over the precise effects and mechanism of ATF3 in hepatic lipid metabolism and AS, especially the expression of low-density LDL receptor. Xu et al. found a direct correlation between hepatocyte ATF3 and AS (17). In mouse liver, the overexpression of ATF3 was shown to induce the scavenger receptor group B type 1 (SR-BI) via P53 and repressed cholesterol 12α-hydroxylase (CYP8B1) through the hepatocyte nuclear factor 4 alpha (HNF4α) pathway, causing increased high-density lipoprotein (HDL) uptake, the inhibition of intestinal fat absorption, and the suppression of AS (17). They also showed that LDL receptor expression and LDL uptake were reduced in ATF3KO HepG2 cells, which suggests that ATF3 expression has a positive effect on LDL metabolism (17). However, ATF3 was reported to lead to the transcriptional repression of LDLR in isolated human liver Sk-Hep1 cells treated with tunicamycin and oligomycin (95). Moreover, Quiroz-Figueroa et al. confirmed that ATF3 mediated the impairment of hepatic LDL catabolism induced by Tribbles pseudokinase 1 (Trib1) depletion via the down-regulation of LDLR, the blunting of which reversed the level of plasma LDL cholesterol and LDLR function in Trib1Δhep mice (96). In summary, the overall role of ATF3 in hepatic lipid metabolism and dyslipidemia is yet to be fully elucidated. further characterization of the PTM and protein interaction of hepatic ATF3 activation will a better understanding of the metabolic syndrome and AS. Besides, there is a wealth of evidence to suggest that ATF3 induction in adipose tissue can affects obesity, but the potential relevance between adipocytic ATF3 expression and dyslipidemia needs to be firmly established (97,98).
Immune response
There is no doubt that immunity and inflammation play crucial roles in AS. Earlier in the article, we described the effects of ATF3 expression in infiltrating macrophages in plaque. However, recent evidence indicates that ATF3 has broader effects in AS-associated immune regulation. In type 1 regulatory T cell, which have been reported to be atheroprotective, ATF3 was shown to be correlated with the regulatory network of anti-inflammatory cytokine IL-10 (95,99). The anti-inflammatory property of ATF3 was found in bone marrow-derived macrophages (97). De Nardo et al. confirmed that HDL suppressed inflammatory response in an ATF3-dependent manner, the activation of later markedly inhibits the TLR-induced production of pro-inflammatory cytokines such as IL-6 and IL-12p40 (97). Elevated level of IL-6 and TNF, but a decreased level of IL-10, have also been found in ATF3 knockdown macrophages, which are associated with the disinhibitory effect of NF-κB P65 via the translocation and phosphorylation at Ser-536 (99). Nus et al. described a type of B-lymphocyte-dependent immune response based on the splenic marginal zone B (MZB) cells, which plays a repressing role in AS. They also confirmed that ATF3 expression in MZB cells inhibits the response of follicular helper T cells partly by suppressing IL-6 expression, which in turn results in protective effects against AS (100). Taken together, these observations suggest that ATF3 mainly acts as suppressor of inflammation and AS development. However, the relationship between ATF3 and the different immune cell subsets involved in AS requires further investigation.
Conclusions
The crucial but rather complicated role of ATF3 in AS has gradually emerged over the past two decades. As a master regulator of vascular homeostasis, metabolic regulation, and immune response, the regulatory diversity of ATF3 in atherogenesis, atherosclerotic lesion progression, and plaque stability is partly attributed to the different responses from 2 promoters, selective expression of ATF3 transcript variants, and a variety of PTM in distinct cell types. Additionally, ATF3 directly affects atherosclerotic lesions by modulating endothelial inflammation, foam cell formation, and vascular remodeling. The expression of ATF3 outside vascular tissue also gets involved in AS by regulating glucolipid metabolism and the inflammatory response. From a forward-looking standpoint, the overall effect of the ATF3 regulatory network on the fate switching and cellular states of VSMCs and macrophages under AS suggest that more attention is merited. Another aspect worthy of deeper investigation is the probable presence and effects of ATF3 in other immune cell subsets correlated with AS. Further, although the diverse effects of ATF3 expression in different tissues and organs once severely restricted the clinical practice of pharmacological intervention targeting ATF3, the latest advances in molecular-targeted therapy make it possible to attenuate atherosclerotic disease via the selective activation or repression of ATF3 in a specific tissue (51). Gaining a better understanding of how ATF3 affects vascular, metabolic, and immune homeostasis could also advance the progress of ATF3-targeted therapy in AS.
Acknowledgments
The authors would like to acknowledge the China Three Gorges University (Yichang, China) for making this review possible.
Funding: This work was supported by National Natural Science Foundation of China (Nos. 82170418, 81770360 and 82070372), Hubei Province’s Outstanding Medical Academic Leader Program.
Footnote
Reporting Checklist: The authors have completed the Narrative Review reporting checklist. Available at https://cdt.amegroups.com/article/view/10.21037/cdt-22-206/rc
Peer Review File: Available at https://cdt.amegroups.com/article/view/10.21037/cdt-22-206/prf
Conflicts of Interest: All authors have completed the ICMJE uniform disclosure form (available at https://cdt.amegroups.com/article/view/10.21037/cdt-22-206/coif). The authors have no conflicts of interest to declare.
Ethical Statement: The authors are accountable for all aspects of the work in ensuring that questions related to the accuracy or integrity of any part of the work are appropriately investigated and resolved.
Open Access Statement: This is an Open Access article distributed in accordance with the Creative Commons Attribution-NonCommercial-NoDerivs 4.0 International License (CC BY-NC-ND 4.0), which permits the non-commercial replication and distribution of the article with the strict proviso that no changes or edits are made and the original work is properly cited (including links to both the formal publication through the relevant DOI and the license). See: https://creativecommons.org/licenses/by-nc-nd/4.0/.
References
- Benjamin EJ, Virani SS, Callaway CW, et al. Heart Disease and Stroke Statistics-2018 Update: A Report From the American Heart Association. Circulation 2018;137:e67-e492. [Crossref] [PubMed]
- Libby P, Buring JE, Badimon L, et al. Atherosclerosis. Nat Rev Dis Primers 2019;5:56. [Crossref] [PubMed]
- Getz GS, Reardon CA. Atherosclerosis: cell biology and lipoproteins. Curr Opin Lipidol 2020;31:286-90. [Crossref] [PubMed]
- Arnett DK, Blumenthal RS, Albert MA, et al. 2019 ACC/AHA Guideline on the Primary Prevention of Cardiovascular Disease: A Report of the American College of Cardiology/American Heart Association Task Force on Clinical Practice Guidelines. Circulation 2019;140:e596-646. [Crossref] [PubMed]
- Libby P. The changing landscape of atherosclerosis. Nature 2021;592:524-33. [Crossref] [PubMed]
- Niu N, Xu S, Xu Y, et al. Targeting Mechanosensitive Transcription Factors in Atherosclerosis. Trends Pharmacol Sci 2019;40:253-66. [Crossref] [PubMed]
- Hai T, Hartman MG. The molecular biology and nomenclature of the activating transcription factor/cAMP responsive element binding family of transcription factors: activating transcription factor proteins and homeostasis. Gene 2001;273:1-11. [Crossref] [PubMed]
- Hai T, Wolfgang CD, Marsee DK, et al. ATF3 and stress responses. Gene Expr 1999;7:321-35. [PubMed]
- Chen BP, Liang G, Whelan J, et al. ATF3 and ATF3 delta Zip. Transcriptional repression versus activation by alternatively spliced isoforms. J Biol Chem 1994;269:15819-26. [Crossref] [PubMed]
- Hai T, Curran T. Cross-family dimerization of transcription factors Fos/Jun and ATF/CREB alters DNA binding specificity. Proc Natl Acad Sci U S A 1991;88:3720-4. [Crossref] [PubMed]
- Rohini M, Haritha Menon A, Selvamurugan N. Role of activating transcription factor 3 and its interacting proteins under physiological and pathological conditions. Int J Biol Macromol 2018;120:310-7. [Crossref] [PubMed]
- Zhu Q, Wang H, Jiang B, et al. Loss of ATF3 exacerbates liver damage through the activation of mTOR/p70S6K/HIF-1α signaling pathway in liver inflammatory injury. Cell Death Dis 2018;9:910. [Crossref] [PubMed]
- Yan F, Wu Y, Liu H, et al. ATF3 is positively involved in particulate matter-induced airway inflammation in vitro and in vivo. Toxicol Lett 2018;287:113-21. [Crossref] [PubMed]
- Qin W, Yang H, Liu G, et al. Activating transcription factor 3 is a potential target and a new biomarker for the prognosis of atherosclerosis. Hum Cell 2021;34:49-59. [Crossref] [PubMed]
- Chen WJ, Lai YJ, Lee JL, et al. CREB/ATF3 signaling mediates indoxyl sulfate-induced vascular smooth muscle cell proliferation and neointimal formation in uremia. Atherosclerosis 2020;315:43-54. [Crossref] [PubMed]
- Wang Y, Gao H, Wang F, et al. Dynamic changes in chromatin accessibility are associated with the atherogenic transitioning of vascular smooth muscle cells. Cardiovasc Res 2022;118:2792-804. [Crossref] [PubMed]
- Xu Y, Li Y, Jadhav K, et al. Hepatocyte ATF3 protects against atherosclerosis by regulating HDL and bile acid metabolism. Nat Metab 2021;3:59-74. [Crossref] [PubMed]
- Hai TW, Liu F, Coukos WJ, et al. Transcription factor ATF cDNA clones: an extensive family of leucine zipper proteins able to selectively form DNA-binding heterodimers. Genes Dev 1989;3:2083-90. [Crossref] [PubMed]
- Deutsch PJ, Hoeffler JP, Jameson JL, et al. Structural determinants for transcriptional activation by cAMP-responsive DNA elements. J Biol Chem 1988;263:18466-72. [Crossref] [PubMed]
- Wolfgang CD, Chen BP, Martindale JL, et al. gadd153/Chop10, a potential target gene of the transcriptional repressor ATF3. Mol Cell Biol 1997;17:6700-7. [Crossref] [PubMed]
- Whitmore MM, Iparraguirre A, Kubelka L, et al. Negative regulation of TLR-signaling pathways by activating transcription factor-3. J Immunol 2007;179:3622-30. [Crossref] [PubMed]
- Lu D, Wolfgang CD, Hai T. Activating transcription factor 3, a stress-inducible gene, suppresses Ras-stimulated tumorigenesis. J Biol Chem 2006;281:10473-81. [Crossref] [PubMed]
- Khuu CH, Barrozo RM, Hai T, et al. Activating transcription factor 3 (ATF3) represses the expression of CCL4 in murine macrophages. Mol Immunol 2007;44:1598-605. [Crossref] [PubMed]
- Li X, Guo M, Cai L, et al. Competitive ubiquitination activates the tumor suppressor p53. Cell Death Differ 2020;27:1807-18. [Crossref] [PubMed]
- Nyunt T, Britton M, Wanichthanarak K, et al. Mitochondrial oxidative stress-induced transcript variants of ATF3 mediate lipotoxic brain microvascular injury. Free Radic Biol Med 2019;143:25-46. [Crossref] [PubMed]
- Ku HC, Cheng CF. Master Regulator Activating Transcription Factor 3 (ATF3) in Metabolic Homeostasis and Cancer. Front Endocrinol (Lausanne) 2020;11:556. [Crossref] [PubMed]
- Hua B, Tamamori-Adachi M, Luo Y, et al. A splice variant of stress response gene ATF3 counteracts NF-kappaB-dependent anti-apoptosis through inhibiting recruitment of CREB-binding protein/p300 coactivator. J Biol Chem 2006;281:1620-9. [Crossref] [PubMed]
- Wang L, Zhu R, Wang J, et al. Nrf2 Activation Enhances Muscular MCT1 Expression and Hypoxic Exercise Capacity. Med Sci Sports Exerc 2020;52:1719-28. [Crossref] [PubMed]
- Pan Y, Chen H, Siu F, et al. Amino acid deprivation and endoplasmic reticulum stress induce expression of multiple activating transcription factor-3 mRNA species that, when overexpressed in HepG2 cells, modulate transcription by the human asparagine synthetase promoter. J Biol Chem 2003;278:38402-12. [Crossref] [PubMed]
- Wang J, Cao Y, Steiner DF. Regulation of proglucagon transcription by activated transcription factor (ATF) 3 and a novel isoform, ATF3b, through the cAMP-response element/ATF site of the proglucagon gene promoter. J Biol Chem 2003;278:32899-904. [Crossref] [PubMed]
- Di Marcantonio D, Martinez E, Kanefsky JS, et al. ATF3 coordinates serine and nucleotide metabolism to drive cell cycle progression in acute myeloid leukemia. Mol Cell 2021;81:2752-2764.e6. [Crossref] [PubMed]
- Kalfon R, Friedman T, Eliachar S, et al. JDP2 and ATF3 deficiencies dampen maladaptive cardiac remodeling and preserve cardiac function. PLoS One 2019;14:e0213081. [Crossref] [PubMed]
- Hu H, Zhang F, Li L, et al. Identification and Validation of ATF3 Serving as a Potential Biomarker and Correlating With Pharmacotherapy Response and Immune Infiltration Characteristics in Rheumatoid Arthritis. Front Mol Biosci 2021;8:761841. [Crossref] [PubMed]
- Lu S, Wang XZ, He C, et al. ATF3 contributes to brucine-triggered glioma cell ferroptosis via promotion of hydrogen peroxide and iron. Acta Pharmacol Sin 2021;42:1690-702. [Crossref] [PubMed]
- Wang L, Liu Y, Du T, et al. ATF3 promotes erastin-induced ferroptosis by suppressing system Xc. Cell Death Differ 2020;27:662-75. [Crossref] [PubMed]
- Tang Y, Pacary E, Fréret T, et al. Effect of hypoxic preconditioning on brain genomic response before and following ischemia in the adult mouse: identification of potential neuroprotective candidates for stroke. Neurobiol Dis 2006;21:18-28. [Crossref] [PubMed]
- Liang G, Wolfgang CD, Chen BP, et al. ATF3 gene. Genomic organization, promoter, and regulation. J Biol Chem 1996;271:1695-701. [Crossref] [PubMed]
- Holtz WA, Turetzky JM, O'Malley KL. Microarray expression profiling identifies early signaling transcripts associated with 6-OHDA-induced dopaminergic cell death. Antioxid Redox Signal 2005;7:639-48. [Crossref] [PubMed]
- Miyazaki K, Inoue S, Yamada K, et al. Differential usage of alternate promoters of the human stress response gene ATF3 in stress response and cancer cells. Nucleic Acids Res 2009;37:1438-51. [Crossref] [PubMed]
- St Germain C, Niknejad N, Ma L, et al. Cisplatin induces cytotoxicity through the mitogen-activated protein kinase pathways and activating transcription factor 3. Neoplasia 2010;12:527-38. [Crossref] [PubMed]
- Zheng S, Abraham C. NF-κB1 inhibits NOD2-induced cytokine secretion through ATF3-dependent mechanisms. Mol Cell Biol 2013;33:4857-71. [Crossref] [PubMed]
- Mathiasen DP, Egebjerg C, Andersen SH, et al. Identification of a c-Jun N-terminal kinase-2-dependent signal amplification cascade that regulates c-Myc levels in ras transformation. Oncogene 2012;31:390-401. [Crossref] [PubMed]
- Underhill-Day N, Heath JK, Oncostatin M. OSM) cytostasis of breast tumor cells: characterization of an OSM receptor beta-specific kernel. Cancer Res 2006;66:10891-901. [Crossref] [PubMed]
- Wang CM, Yang WH, Cardoso L, et al. Forkhead Box Protein P3 (FOXP3) Represses ATF3 Transcriptional Activity. Int J Mol Sci 2021;22:11400. [Crossref] [PubMed]
- Klingenberg R, Gerdes N, Badeau RM, et al. Depletion of FOXP3+ regulatory T cells promotes hypercholesterolemia and atherosclerosis. J Clin Invest 2013;123:1323-34. [Crossref] [PubMed]
- Ruotsalainen AK, Inkala M, Partanen ME, et al. The absence of macrophage Nrf2 promotes early atherogenesis. Cardiovasc Res 2013;98:107-15. [Crossref] [PubMed]
- Kha ML, Hesse L, Deisinger F, et al. The antioxidant transcription factor Nrf2 modulates the stress response and phenotype of malignant as well as premalignant pancreatic ductal epithelial cells by inducing expression of the ATF3 splicing variant ΔZip2. Oncogene 2019;38:1461-76. [Crossref] [PubMed]
- Balasubramanian MN, Shan J, Kilberg MS. Dynamic changes in genomic histone association and modification during activation of the ASNS and ATF3 genes by amino acid limitation. Biochem J 2013;449:219-29. [Crossref] [PubMed]
- Tran NQV, Nguyen AN, Takabe K, et al. Pre-treatment with amitriptyline causes epigenetic up-regulation of neuroprotection-associated genes and has anti-apoptotic effects in mouse neuronal cells. Neurotoxicol Teratol 2017;62:1-12. [Crossref] [PubMed]
- Chüeh AC, Tse JWT, Dickinson M, et al. ATF3 Repression of BCL-XL Determines Apoptotic Sensitivity to HDAC Inhibitors across Tumor Types. Clin Cancer Res 2017;23:5573-84. [Crossref] [PubMed]
- Duncan RM, Reyes L, Moats K, et al. ATF3 Coordinates Antitumor Synergy between Epigenetic Drugs and Protein Disulfide Isomerase Inhibitors. Cancer Res 2020;80:3279-91. [Crossref] [PubMed]
- Yuan L, Mu P, Huang B, et al. EGR1 is essential for deoxynivalenol-induced G2/M cell cycle arrest in HepG2 cells via the ATF3ΔZip2a/2b-EGR1-p21 pathway. Toxicol Lett 2018;299:95-103. [Crossref] [PubMed]
- Pollack BP, Sapkota B, Boss JM. Ultraviolet radiation-induced transcription is associated with gene-specific histone acetylation. Photochem Photobiol 2009;85:652-62. [Crossref] [PubMed]
- Li L, Sun RM, Jiang GQ. ATF3 Demethylation Promotes the Transcription of ARL4C, Which Acts as a Tumor Suppressor in Human Breast Cancer. Onco Targets Ther 2020;13:3467-76. [Crossref] [PubMed]
- Pan YX, Chen H, Kilberg MS. Interaction of RNA-binding proteins HuR and AUF1 with the human ATF3 mRNA 3'-untranslated region regulates its amino acid limitation-induced stabilization. J Biol Chem 2005;280:34609-16. [Crossref] [PubMed]
- He F, Zhou M, Yu T, et al. Sublytic C5b-9 triggers glomerular mesangial cell apoptosis in rat Thy-1 nephritis via Gadd45 activation mediated by Egr-1 and p300-dependent ATF3 acetylation. J Mol Cell Biol 2016;8:477-91. [Crossref] [PubMed]
- Li G, Zhang J, Liu D, et al. Identification of Hub Genes and Potential ceRNA Networks of Diabetic Nephropathy by Weighted Gene Co-Expression Network Analysis. Front Genet 2021;12:767654. [Crossref] [PubMed]
- Zhu Y, He D, Bo H, et al. The MRVI1-AS1/ATF3 signaling loop sensitizes nasopharyngeal cancer cells to paclitaxel by regulating the Hippo-TAZ pathway. Oncogene 2019;38:6065-81. [Crossref] [PubMed]
- Weng YL, Wang X, An R, et al. Epitranscriptomic m6A Regulation of Axon Regeneration in the Adult Mammalian Nervous System. Neuron 2018;97:313-325.e6. [Crossref] [PubMed]
- Liu X, Yuan J, Zhang X, et al. ATF3 Modulates the Resistance of Breast Cancer Cells to Tamoxifen through an N6-Methyladenosine-Based Epitranscriptomic Mechanism. Chem Res Toxicol 2021;34:1814-21. [Crossref] [PubMed]
- Wang CM, Brennan VC, Gutierrez NM, et al. SUMOylation of ATF3 alters its transcriptional activity on regulation of TP53 gene. J Cell Biochem 2013;114:589-98. [Crossref] [PubMed]
- Zhang ZB, Ruan CC, Chen DR, et al. Activating transcription factor 3 SUMOylation is involved in angiotensin II-induced endothelial cell inflammation and dysfunction. J Mol Cell Cardiol 2016;92:149-57. [Crossref] [PubMed]
- Wang CM, Yang WH. Loss of SUMOylation on ATF3 inhibits proliferation of prostate cancer cells by modulating CCND1/2 activity. Int J Mol Sci 2013;14:8367-80. [Crossref] [PubMed]
- Epanchintsev A, Costanzo F, Rauschendorf MA, et al. Cockayne's Syndrome A and B Proteins Regulate Transcription Arrest after Genotoxic Stress by Promoting ATF3 Degradation. Mol Cell 2017;68:1054-1066.e6. [Crossref] [PubMed]
- Vu TTM, Mitchell DC, Gygi SP, et al. The Arg/N-degron pathway targets transcription factors and regulates specific genes. Proc Natl Acad Sci U S A 2020;117:31094-104. [Crossref] [PubMed]
- Mishra R, Lahon A, Banerjea AC. Dengue Virus Degrades USP33-ATF3 Axis via Extracellular Vesicles to Activate Human Microglial Cells. J Immunol 2020;205:1787-98. [Crossref] [PubMed]
- Nawa T, Nawa MT, Adachi MT, et al. Expression of transcriptional repressor ATF3/LRF1 in human atherosclerosis: colocalization and possible involvement in cell death of vascular endothelial cells. Atherosclerosis 2002;161:281-91. [Crossref] [PubMed]
- Aung HH, Lame MW, Gohil K, et al. Induction of ATF3 gene network by triglyceride-rich lipoprotein lipolysis products increases vascular apoptosis and inflammation. Arterioscler Thromb Vasc Biol 2013;33:2088-96. [Crossref] [PubMed]
- Zhang C, Kawauchi J, Adachi MT, et al. Activation of JNK and transcriptional repressor ATF3/LRF1 through the IRE1/TRAF2 pathway is implicated in human vascular endothelial cell death by homocysteine. Biochem Biophys Res Commun 2001;289:718-24. [Crossref] [PubMed]
- Teasdale JE, Hazell GG, Peachey AM, et al. Cigarette smoke extract profoundly suppresses TNFα-mediated proinflammatory gene expression through upregulation of ATF3 in human coronary artery endothelial cells. Sci Rep 2017;7:39945. [Crossref] [PubMed]
- McDonald AI, Shirali AS, Aragón R, et al. Endothelial Regeneration of Large Vessels Is a Biphasic Process Driven by Local Cells with Distinct Proliferative Capacities. Cell Stem Cell 2018;23:210-225.e6. [Crossref] [PubMed]
- Chen CC, Hong HJ, Hao WR, et al. Nicorandil prevents doxorubicin-induced human umbilical vein endothelial cell apoptosis. Eur J Pharmacol 2019;859:172542. [Crossref] [PubMed]
- Yang S, Yuan HQ, Hao YM, et al. Macrophage polarization in atherosclerosis. Clin Chim Acta 2020;501:142-6. [Crossref] [PubMed]
- Suganami T, Yuan X, Shimoda Y, et al. Activating transcription factor 3 constitutes a negative feedback mechanism that attenuates saturated Fatty acid/toll-like receptor 4 signaling and macrophage activation in obese adipose tissue. Circ Res 2009;105:25-32. [Crossref] [PubMed]
- Sha H, Zhang D, Zhang Y, et al. ATF3 promotes migration and M1/M2 polarization of macrophages by activating tenascin-C via Wnt/β-catenin pathway. Mol Med Rep 2017;16:3641-7. [Crossref] [PubMed]
- Li D, Yan M, Sun F, et al. miR-498 inhibits autophagy and M2-like polarization of tumor-associated macrophages in esophageal cancer via MDM2/ATF3. Epigenomics 2021;13:1013-30. [Crossref] [PubMed]
- Gold ES, Ramsey SA, Sartain MJ, et al. ATF3 protects against atherosclerosis by suppressing 25-hydroxycholesterol-induced lipid body formation. J Exp Med 2012;209:807-17. [Crossref] [PubMed]
- Luo H, Wang J, Qiao C, et al. ATF3 Inhibits Tenascin-C-induced Foam Cell Formation in LPS-Stimulated THP-1 Macrophages by Suppressing TLR-4. J Atheroscler Thromb 2015;22:1214-23. [Crossref] [PubMed]
- Nguyen HCB, Adlanmerini M, Hauck AK, et al. Dichotomous engagement of HDAC3 activity governs inflammatory responses. Nature 2020;584:286-90. [Crossref] [PubMed]
- Choe N, Kwon DH, Ryu J, et al. miR-27a-3p Targets ATF3 to Reduce Calcium Deposition in Vascular Smooth Muscle Cells. Mol Ther Nucleic Acids 2020;22:627-39. [Crossref] [PubMed]
- Liu X, Liu X, Li M, et al. Mechanical Stretch Induces Smooth Muscle Cell Dysfunction by Regulating ACE2 via P38/ATF3 and Post-transcriptional Regulation by miR-421. Front Physiol 2021;11:540591. [Crossref] [PubMed]
- Edgar L, Akbar N, Braithwaite AT, et al. Hyperglycemia Induces Trained Immunity in Macrophages and Their Precursors and Promotes Atherosclerosis. Circulation 2021;144:961-82. [Crossref] [PubMed]
- Hartman MG, Lu D, Kim ML, et al. Role for activating transcription factor 3 in stress-induced beta-cell apoptosis. Mol Cell Biol 2004;24:5721-32. [Crossref] [PubMed]
- Zmuda EJ, Viapiano M, Grey ST, et al. Deficiency of Atf3, an adaptive-response gene, protects islets and ameliorates inflammation in a syngeneic mouse transplantation model. Diabetologia 2010;53:1438-50. [Crossref] [PubMed]
- Gurzov EN, Barthson J, Marhfour I, et al. Pancreatic β-cells activate a JunB/ATF3-dependent survival pathway during inflammation. Oncogene 2012;31:1723-32. [Crossref] [PubMed]
- Duprez J, Jonas JC. Role of activating transcription factor 3 in low glucose- and thapsigargin-induced apoptosis in cultured mouse islets. Biochem Biophys Res Commun 2011;415:294-9. [Crossref] [PubMed]
- Kim S, Song NJ, Bahn G, et al. Atf3 induction is a therapeutic target for obesity and metabolic diseases. Biochem Biophys Res Commun 2018;504:903-8. [Crossref] [PubMed]
- Cheng CF, Ku HC, Cheng JJ, et al. Adipocyte browning and resistance to obesity in mice is induced by expression of ATF3. Commun Biol 2019;2:389. [Crossref] [PubMed]
- Kalfon R, Koren L, Aviram S, et al. ATF3 expression in cardiomyocytes preserves homeostasis in the heart and controls peripheral glucose tolerance. Cardiovasc Res 2017;113:134-46. [Crossref] [PubMed]
- Kim JY, Park KJ, Hwang JY, et al. Activating transcription factor 3 is a target molecule linking hepatic steatosis to impaired glucose homeostasis. J Hepatol 2017;67:349-59. [Crossref] [PubMed]
- Tsai WW, Matsumura S, Liu W, et al. ATF3 mediates inhibitory effects of ethanol on hepatic gluconeogenesis. Proc Natl Acad Sci U S A 2015;112:2699-704. [Crossref] [PubMed]
- Lee YS, Sasaki T, Kobayashi M, et al. Hypothalamic ATF3 is involved in regulating glucose and energy metabolism in mice. Diabetologia 2013;56:1383-93. [Crossref] [PubMed]
- Lacy M, Atzler D, Liu R, et al. Interactions between dyslipidemia and the immune system and their relevance as putative therapeutic targets in atherosclerosis. Pharmacol Ther 2019;193:50-62. [Crossref] [PubMed]
- Chou CL, Li CH, Lin H, et al. Role of activating transcription factor 3 in fructose-induced metabolic syndrome in mice. Hypertens Res 2018;41:589-97. [Crossref] [PubMed]
- Lim JH, Lee HJ, Pak YK, et al. Organelle stress-induced activating transcription factor-3 downregulates low-density lipoprotein receptor expression in Sk-Hep1 human liver cells. Biol Chem 2011;392:377-85. [Crossref] [PubMed]
- Quiroz-Figueroa K, Vitali C, Conlon DM, et al. TRIB1 regulates LDL metabolism through CEBPα-mediated effects on the LDL receptor in hepatocytes. J Clin Invest 2021;131:e146775. [Crossref] [PubMed]
- De Nardo D, Labzin LI, Kono H, et al. High-density lipoprotein mediates anti-inflammatory reprogramming of macrophages via the transcriptional regulator ATF3. Nat Immunol 2014;15:152-60. [Crossref] [PubMed]
- Zhao Q, Luo YF, Tian M, et al. Activating transcription factor 3 involved in Pseudomonas aeruginosa PAO1-induced macrophage senescence. Mol Immunol 2021;133:122-7. [Crossref] [PubMed]
- Zhang H, Madi A, Yosef N, et al. An IL-27-Driven Transcriptional Network Identifies Regulators of IL-10 Expression across T Helper Cell Subsets. Cell Rep 2020;33:108433. [Crossref] [PubMed]
- Nus M, Sage AP, Lu Y, et al. Marginal zone B cells control the response of follicular helper T cells to a high-cholesterol diet. Nat Med 2017;23:601-10. [Crossref] [PubMed]