M3 subtype of muscarinic acetylcholine receptor inhibits cardiac fibrosis via targeting microRNA-29b/beta-site app cleaving enzyme 1 axis
Highlight box
Key findings
• Choline produces the anti-fibrotic effects through activating M3 subtype of muscarinic acetylcholine receptor (M3 receptor) to upregulate microRNA-29b (miR-29b) in cardiac.
• Beta-site app cleaving enzyme 1 (BACE1), a downstream target gene of miR-29b, is involved in the anti-fibrotic effects of activating of M3 receptor.
What is known and what is new?
• Anti-fibrotic effects of miR-29b are known.
• The anti-fibrotic effects produced by activation of M3 receptor is mediated by upregulation of miR-29b, and we identified BACE1 as a novel target for myocardial fibrosis that could be involved in this anti-fibrotic pathway.
What is the implication, and what should change now?
• This further illustrates the role of activating M3 receptor in exerting anti-fibrotic effects and provides ideas for clinical treatment.
• Further explore the role of BACE1, the target identified in this study, in the progression of myocardial fibrosis and heart failure.
Introduction
Background
Cardiovascular disease is one of the main causes of hospitalization and mortality, and is a large public health burden in the world (1). Cardiac fibrosis is a common pathophysiological process occurring along with a variety of diseases, including arrhythmia (2), heart failure (3) and sudden cardiac death (4). It is a pathological change from cardiac compensation to decompensation and can be caused by numerous factors. The fibroblast-myofibroblast transformation is a hallmark change in myocardial fibrosis, characterized by the secretion of extracellular matrix proteins including collagen deposition. In myofibroblasts, many endoplasmic reticulum form aggregates and express contractile proteins such as α-smooth muscle actin (α-SMA) and extracellular matrix proteins (5). Subsequently, myofibroblasts undergo migration and contraction, promoting the development of cardiac fibrosis. Several signaling pathways have been reported to be involved in the development of cardiac fibrosis, including transforming growth factor -β (TGF-β) (6), mitogen-activated protein kinase (MAPK) (7), wingless/integrated (Wnt) (8), renin-angiotensin-aldosterone system (RAAS) (9) and other signaling pathways.
Muscarinic acetylcholine receptors (M receptors) belong to the heterotrimeric G protein-coupled receptors (GPCR) family and can be bound by endogenous agonist acetylcholine (10), as well as choline (11), to excite parasympathetic nerve endings and act differently depending on the downstream effector. M3 subtype of muscarinic acetylcholine receptor (M3 receptor) is one of the M receptor subtypes, which is involved in the development of a variety of cardiovascular diseases. In ischemic arrhythmias, choline protects against myocardial ischemia-induced arrhythmias in rats through activating the M3 receptor, mainly by reducing intracellular calcium overload (12). Activation of M3 receptor can also prevent Angiotensin II-induced cardiac hypertrophy by inhibiting reactive oxygen species (ROS)-mediated p38 MAPK activation and regulating Ca2+-mediated calcineurin signal transduction pathway (13). The above results indicate the important role of M3 receptor in cardiomyocytes. Our pilot study revealed that M3 receptor was expressed in cardiac fibroblasts in almost the same abundance as cardiomyocytes under basal conditions, and activation of M3 receptor inhibited cardiac fibrosis and reduced collagen secretion in mice by modulating the classical pathways of fibrosis, TGF-β1/Smad2/3 and p38 MAPK signaling pathways (14).
In this study, we used Kunming mice as the research subjects. The mice we used were characterized by easy rearing, high reproduction rate, easy accessibility, small inter-individual differences, and high disease resistance and adaptability, which improved the efficiency of our experiments and facilitated the observation of the results of parallel experiments. Meanwhile, they’re close to humans in terms of biological evolution, with similar tissue organs and cellular functions and good genetic homology. Cells in primary culture have just been separated from living tissues and are closer to the morphology, structure and functional activities of the original tissues in the organism, and are able to represent signal transduction in vivo very closely, thus allowing for better cellular disease models to be established through primary cells.
Rationale and knowledge gap
M3 receptor belongs to the class of GPCRs that mediate many physiological responses in eukaryotes, and it is not clear whether new molecules are involved in this anti-fibrotic process after the activation of M3 receptor, nor what its upstream and downstream factors are. We explored new regulatory factors and targets of this process in the present study.
Micro-RNAs (miRNAs) belong to a highly conserved, endogenous non-coding RNA family with a short length of approximately 22 nucleotides, and these molecules post-transcriptionally regulate gene expression by binding to the 3'-untranslated region of targeted messenger RNAs by partial complementarity (15). MicroRNA-29 (miR-29) forms a miRNA seed family, which consists of several members including miR-29a, miR-29b and miR-29c (16). Mature miR-29 is highly conserved in human, mouse and rat. MiR-29b is involved in a variety of pathways that regulate cardiovascular disease, and it may be useful for treatment or diagnostic of diseases (17). While a part of roles of miR-29b remain poorly understood, recent studies have provided important insights into the relevance of miR-29b to fibrotic diseases, such as the miR-29b plays a protective role in renal fibrosis (18), liver fibrosis (19), and pulmonary fibrosis (20). Moreover, miR-29b plays an important role in myocardial fibrosis (21).
M3 receptor can stimulate the nuclear factor kappa-B (NF-κB) signaling pathway to inhibit the expression of miR-376b-5p and produce a cardioprotective effect (22). M3 receptor overexpression shortens cardiac action potential duration through downregulation of miR-1 and prevents arrhythmias in mice with myocardial ischemia-reperfusion (23). The connection between M3 receptor and miRNAs prompted us to propose that M3 receptor might regulate miRNA to alter cardiac function and vice versa, miRNAs might act as downstream factors mediating the beneficial effects of M3 receptor.
Due to the important role of miR-29b in the fibrotic process, and the unexplored relationship between the M3 receptor and miR-29b, we investigated whether miR-29b was involved in the anti-fibrotic effect of M3 receptor. Meanwhile, miRNAs usually act on targets to play their functions, whether a new target of miR-29b in myocardial fibrosis can be found is another purpose of this paper. It also provides new insights for clinical studies of myocardial fibrosis.
Objective
The goal of the present study was to explore the potential connection between M3 receptor and miRNAs in the setting of cardiac fibrosis with a focus on miR-29b that is known to be a regulator of cardiac fibrosis. We assumed that the expression of miR-29b may be raised after activating the M3 receptor, and the downstream target gene was controlled to affect fibrosis. We present this article in accordance with the ARRIVE reporting checklist (available at https://cdt.amegroups.com/article/view/10.21037/cdt-23-309/rc).
Methods
Neonatal mouse cardiac fibroblasts isolation and culture
Neonatal Kunming mice (1–3 days old) were purchased from the Animal Center of the Second Affiliated Hospital of Harbin Medical University (Harbin, China). The license number is SCXK(HeilongJiang)2019-001. The study was reviewed and approved by the Ethics Committee of the Second Affiliated Hospital of Harbin Medical University (Approval number: Ky2016-103. Approval time: 9 March 2016), in compliance with the “Laboratory animal—Guideline for ethical review of animal welfare” (GB/T 35892-2018) national guidelines for the care and use of animals. A protocol was prepared before the study without registration. We strive to use fewer animals required by experiments by improving experimental methods, and obtain more data with the minimum quantity of animals. Moreover, we painlessly execute animals and then take their hearts for in vitro studies, which is a relative substitute for live animals. Throughout the experiment, we treat experimental animals kindly, respect animal life, and thank animals for the effort for our human scientific research. No exclusion criteria was set during the experiment. The hearts of randomly selected neonatal mice were dissected and cut into 2–3 pieces and placed in Dulbecco’s modified Eagle’s medium (DMEM, HyClone, USA). The preparation was transferred into 50 mL centrifuge tubes, followed by the addition of prewarmed 0.25% trypsin for cleaning and digestion. The collected cell suspension was filtered, and cells were obtained by centrifugation at 1,000 r/min for 5 min. The cells were resuspended in DMEM containing 10% fetal bovine serum (FBS) and 5% penicillin/streptomycin and then incubated in Petri dishes (60 mm) or other specifications of culture plates. The cells were then cultured under a condition of 5% CO2 and 95% air at 37 ℃ for 1.5 h to allow cardiac fibroblasts to adhere to the bottom of the Petri dishes and leave the isolated cardiac myocytes and other cells suspended. The cardiac fibroblasts were cultured under the same conditions for 48 h before being examined for morphology and density. The cells were incubated with 3 nM of 4-diphenylacetoxy-N-methyl-piperidine methiodide (4-DAMP) (Abcam, USA), 1 mM of choline (Sigma, USA) or 20 ng/mL of TGF-β1 (PeproTech, USA) as to be specified in the appropriate sections as described previously (14).
Transfection procedures
Prior to transfection, the cardiac fibroblasts were confirmed to be in good condition under a microscope and devoid of contamination, followed by incubation in fresh culture medium for 4–6 h. The cells were then transfected with 50 nM of miR-29b mimics or inhibitor using Opti-MEM (Invitrogen, USA) and X-treme GENE siRNA transfection reagent (Roche, Penzberg Germany) for 6 h according to the manufacturer’s protocols. Next, the cells were kept in the medium containing 10% bovine serum. In separate experiments, cells were transfected with 1 µg/mL beta-site app cleaving enzyme 1 (BACE1) plasmid or empty plasmid (pcMV3-C-GFPSpark) through Lipofectamine 2000 (Invitrogen, USA) and X-treme for 6 h. The transfected cardiac fibroblasts were incubated for 24 h and then treated with drugs according to the previous experimental procedure (14). Briefly, the cardiac fibroblasts were pretreated with 3 nM 4-DAMP in the presence of 1 mM choline for 1 h and then incubated with 20 ng/mL TGF-β1 for 48 h. The sequence of miR-29b mimics was 5'-AACACUGAUUUCAAAUGGUGCUA-3' and the sequence of miR-29b inhibitor was 5'-UAGCACCAUUUGAAAUCAGUGUU-3'. The negative control sequence was 5'-UCUACUCUUUCUAGGAGGUUGUGA-3' and all these constructs were purchased from General Biosystems, Inc. (Anhui, China). BACE1 plasmid and empty plasmid were constructed by Sino Biological Inc. (Beijing, China).
Immunofluorescence staining
Cardiac fibroblasts were inoculated onto sterile coverslips in 24-well culture plates and treated accordingly as described in the transfection procedure. After treatment, coverslips were washed three times with cold phosphate buffered saline (PBS) and fixed with 4% paraformaldehyde for 30 min. The coverslips were washed three times again with cold PBS and then permeabilized with 4 mL PBS including bovine serum albumin 0.04 g and Triton X-100 10 µL for 20 min. Goat serum (Solarbio, Beijing, China) was mixed with PBS in an equal volume to block, and the cardiac fibroblasts were incubated at 37 ℃ for 1 h. Subsequently, the cells were incubated with collagen I antibody (Proteintech, Wuhan, China, 1:250) or α-SMA antibody (Abcam, USA, 1:250) at 4 ℃ overnight, followed by incubation with fluorescein isothiocyanate (FITC)-conjugated goat anti-rabbit IgG secondary antibody (ZSGB-Bio, China, 1:500) in the dark at room temperature for 1 h. DAPI (Beyotime, Haimen, China) was added to stain the nuclei for 5 min after washing with PBS, and all steps were protected from light. Images were taken with a laser scanning confocal microscope (Olympus, Fluoview1000, Tokyo, Japan).
Western blot
Cells were rinsed with PBS and lysed using Radio Immunoprecipitation Assay Lysis buffer (RIPA) (Beyotime, Jiangsu, China) supplemented with protease inhibitor mixture. Protein concentration was determined using the Bicinchoninic Acid (BCA) Protein Assay Kit (Beyotime, Shanghai, China) according to the manufacturer’s instructions. Configured the system with RIPA and sodium dodecyl sulfate-polyacrylamide gel electrophoresis (SDS-PAGE) 5× Loading Buffer (Beyotime, Jiangsu, China). The protein sample (70 µg) was fractionated by SDS-PAGE (10% polyacrylamide gel) and transferred to a nitrocellulose membrane. The nitrocellulose membranes were blocked in 5% nonfat milk for 2 h and then incubated with primary antibodies at 4 ℃ in the refrigerator overnight. Primary antibodies included collagen I (1:1,000), α-SMA (1:1,000), CTGF (1:500), BACE1 (1:1,000), and glyceraldehyde-3-phosphate dehydrogenase (GAPDH, 1:1,000). Antibodies against collagen I and CTGF were purchased from Proteintech (Wuhan, China). Antibody against α-SMA was purchased from Abcam (USA) and antibody against BACE1 was purchased from ABclonal (Hubei, China). Antibody against GAPDH was purchased from ZSGB-Bio (China). The membranes were washed three times with PBS-Tween (0.5%), secondary antibodies were used to incubate the membranes in the dark at room temperature for 1 h, and then scanned to capture blot band images with Imaging System (LI-COR Biosciences, Lincoln, NE, USA).
Quantitative real-time reverse transcription polymerase chain reaction (qRT-PCR)
Total RNA from cardiac fibroblasts was extracted using TRIzol™ Reagent (Thermo, USA) according to the manufacturer’s protocol. RNA concentration was determined by Nanodrop 2000 and was reverse transcribed into complementary DNA. Quantification of miR-29b was done by LC480 Real-time PCR system (Roche, USA) using SYBR Green PCR Master Mix (Roche, USA) PCR cycling protocol: 95 ℃ for 10 min, 40 cycles at 95 ℃ for 15 s, 60 ℃ for 30 s and 72 ℃ for 30 s. U6 was used as an internal control and the amount of miR-29b was calculated according to 2–ΔΔCt. The sequences of primers used in this study were: miR-29b, forward: 5'-GCGGTAGCACCATTTGAAATCAGT-3' and reverse: 5'-ATCCAGTGCAGGGTCCGAGG-3'; U6 forward: 5'-GCTTCGGCACATATACTAAAAT-3' and reverse: 5'-CGCTTCACGAATTTGCGTGTCAT-3'.
Scratch test
Cardiac fibroblasts were plated in a 6-well plate, incubated in DMEM containing 10% FBS and 5% penicillin/streptomycin. The cells were cultured under a condition of 5% CO2 and 95% air at 37 ℃ for 48 h before the culture medium was changed to DMEM for 4–6 h. The cells were wounded by scratching using a 10 µL tip, and non-adherent cells were washed with culture medium. Transfection reagent, choline and TGF-β1 treatment were carried out as described in transfection procedures and photographs were taken under a microscope immediately. Photographs were taken at 24 and 48 h after TGF-β1 treatment. The percentages of wound closure area of cell scratches over time were determined using ImageJ software.
Transwell migration assay
Transwell chambers with 8-µm pore size membranes (Corning Life Sciences, Lowell, MA, USA) were used to detect the migration of cardiac fibroblasts. The transfected cells were harvested and reconstituted in serum-free buffer at 5×105 cells/mL, and 100 µL of the cell suspension was taken and added to the chambers. Meanwhile, 600 µL of DMEM containing 10% FBS, TGF-β1, and choline was added to the lower chamber. After incubation, the chamber was cleaned with PBS and fixed with 4% paraformaldehyde for 30 min, followed by staining with crystal violet staining solution for 20 min. The chambers were examined under a microscope.
Statistical analysis
The different stages of the experiment (allocation period, experiment implementation) were handled by different person. Anyone who randomized the experiment by labeled numbers only, without knowing what kind of treatment each group received. Results the evaluation and data analysis results were entered according to the corresponding numbers of each group by another person. GraphPad Prism 9.0 was used for data analysis. The experimental data are presented as the mean ± SEM. One-way analysis of variance (ANOVA) followed by a post hoc Tukey test for multiple group comparisons. Statistical significance was considered when a value of P was <0.05.
Results
Activation of M3 receptor by choline upregulates miR-29b and downregulates fibrotic markers in cardiac fibroblasts
Previous study has demonstrated that choline possesses anti-fibrotic properties through inhibiting the TGF-β1/Smad and p38 MAPK pathways upon activating M3 receptor (14). However, how M3 receptor activation leads to fibrosis inhibition remains elusive. To this end, we detected the expression of miR-29b in cardiac fibroblasts with different treatment conditions. The results showed the level of miR-29b was downregulated in cardiac fibroblasts induced by TGF-β1 (P=0.0389), whereas upregulated after further addition of choline (P=0.0001), and 4-DAMP, a competitive antagonist of M3 receptor reversed the effect of choline (P=0.0013) (Figure 1A). Meanwhile, we detected that the administration of choline and 4-DAMP under physiological conditions did not affect the expression of miR-29b (Figure S1), which may be related to other mechanisms. We also detected the expression of M3 receptor when transfected with miR-29b mimics and miR-29b inhibitor. Transfection efficiency of miR-29b mimics (Figure S2A) and miR-29b inhibitor (Figure S2B) were shown in Figure S2. There was no effect on the protein level of M3 receptor when transfected with miR-29 mimics or miR-29b inhibitor (Figure S3), so we suggested that miR-29b may play a role in myocardial fibrosis as a downstream of the M3 receptor. These data suggested that miR-29b might be involved in the anti-fibrotic effect afforded by choline via activating the M3 receptor when myocardial fibrosis occurs.
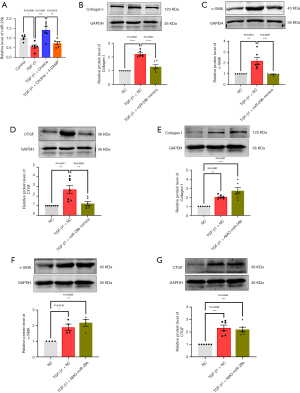
We then overexpressed or inhibited the expression of miR-29b in cardiac fibroblasts to detected the expression of cardiac fibrosis markers such as collagen I, α-SMA and CTGF. The primary endpoint of this study was defined as the protein level of collagen I, α-SMA and CTGF. Compared with the negative control group (NC), the TGF-β1 group showed high expression of collagen I (P<0.0001), α-SMA (P=0.0010), and CTGF (P=0.0011), whereas miR-29b overexpression reversed such an abnormal expression upregulation of collagen I (P<0.0001), α-SMA (P=0.0007), and CTGF (P=0.0038) (Figure 1B-1D), and at the same time, the inhibition of miR-29b expression did not have such an effect on the expression of collagen I, α-SMA, and CTGF (Figure 1E-1G).
MiR-29b mediates the anti-fibrotic action of M3 receptor activation in cardiac fibroblasts
The above results suggested that miR-29b might act as an anti-fibrotic miRNA downstream of the M3 receptor. To clarify this thought, we performed the following experiments. The primary endpoint of this study was defined as the protein level of collagen I, α-SMA and CTGF. First, we demonstrated that choline reversed the overproduction of collagen I protein in the presence of TGF-β1 (P=0.0425) (Figure 2A). Notably, the anti-fibrotic effect of choline was counteracted by miR-29b inhibitor in cardiac fibroblasts (P=0.004), but not by the NC construct (P>0.99) (Figure 2A). Similar results were observed with α-SMA (P=0.0001) and CTGF proteins (P=0.0185) (Figure 2B,2C). This further confirmed the importance of miR-29b in the anti-fibrotic effect of M3 receptor.
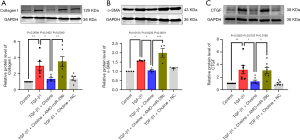
Second, we used immunofluorescence to detect the changes of expression and subcellular distribution of collagen I and α-SMA in cardiac fibroblasts under different treatment conditions. The results uncovered that the expression of collagen I and α-SMA of cardiac fibroblasts which stimulated by TGF-β1 were increased, as indicated by the enhanced fluorescence intensity (P<0.0001). α-SMA served as an indicator of fibroblast-myofibroblast transition, demonstrating that TGF-β1 can promote the conversion of fibroblasts to myofibroblasts. Choline abrogated TGF-β1-induced upregulation of collagen I and α-SMA (P<0.0001), and these beneficial effects of choline were mitigated after transfection with miR-29b inhibitor (P<0.0001) (Figure 3A-3D). We also verified whether overexpression of miR-29b could affect the level of collagen I and α-SMA when M3 receptor was inhibited by 4-DAMP under model conditions. It could be seen that in agreement with previous results, 4-DAMP reversed the effects of choline and increased the expression of collagen I (P=0.0233) and α-SMA (P=0.0026). These were counteracted by miR-29b mimics, which decreased the level of collagen I (P=0.0097) and α-SMA (P=0.0003) (Figure 3E-3H).
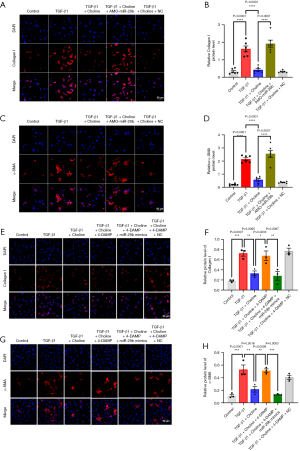
Third, activation of fibroblasts promotes cell proliferation and collagen secretion, so we examined the effects of miR-29b and M3 receptor on the proliferative and migratory capacity of cardiac fibroblasts by two different methods. In the first set of experiments, we used the scratch test to measure the migratory ability of cardiac fibroblasts. We found that TGF-β1 promoted the migration of cardiac fibroblasts (P=0.0001), which was inhibited by choline (P=0.0094), indicating the anti-fibrotic effect of the M3 receptor. Transfection of miR-29b inhibitor eliminated the protective effect of choline (P=0.0095) and aggravated the migration of cardiac fibroblasts (Figure 4A,4B). In the second set of experiments, we used the transwell assay to measure the migratory and invasive ability of cardiac fibroblasts. The results showed that the migration/invasion suppressing effects of choline were abolished following a knockdown of miR-29b by its antisense inhibitor (P=0.0062) (Figure 4C,4D). These consistent results suggested that miR-29b inhibitor promoted cardiac fibrosis even while the M3 receptor had been activated.
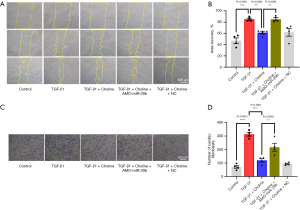
BACE1 participates in the anti-fibrotic effect by activating M3 receptor
It has been reported that miR-29b can fight against fibrotic diseases by directly targeting collagen I and collagen III (24), and in the present study, we screened for new targets of miR-29b against fibrosis. Four online prediction websites for miRNA targets were used, including miRDB (http://www.mirdb.org/), miRTarBase (https://mirtarbase.cuhk.edu.cn/), TargetScan (http://www.targetscan.org/), and PicTar (https://pictar.mdc-berlin.de/), and Venn diagrams were drawn. A total of 19 target genes were identified common to the four databases mentioned above. Through literature review, we screened the target genes based on their expression, abundance in the heart and a good conservation among species. This procedure led us to focus on BACE1 as a new target gene of miR-29b for studying its anti-fibrotic properties (Figure 5A). Sequence alignment revealed multiple binding sites at the 3' non-coding region of BACE1 gene for miR-29b seed site (Figure 5B).
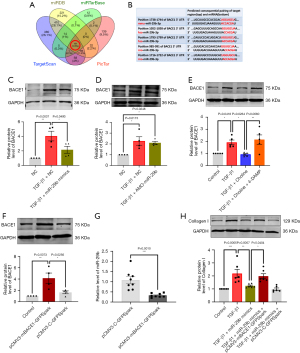
We next experimentally established the targeting relationship between miR-29b and BACE1. Western blot analysis showed that the protein level of BACE1 was increased in the presence of TGF-β1 (P=0.0037) but was reduced after transfection of miR-29b mimics (P=0.0493) (Figure 5C). Meanwhile, inhibition of miR-29b did not affect the effects of TGF-β1 (Figure 5D). We further detected the expression of BACE1 in choline or 4-DAMP treated cardiac fibroblasts. We found that TGF-β1-induced increase in the protein level of BACE1 was mitigated in cells treated with choline (P=0.0264), on the other hand, 4-DAMP, a competitive antagonist of M3 receptor, reversed the effect of choline (P=0.0060) (Figure 5E). We then overexpressed BACE1 by transfecting the cells with the plasmid carrying BACE1 for overexpression. The transfection efficiency of the plasmid was shown in Figure 5F. And we found that overexpression of BACE1 reduced the level of miR-29b (P=0.0010) (Figure 5G). Moreover, overexpression of BACE1 eliminated the anti-fibrotic effect elicited by miR-29b mimics (P=0.0404) and restored the expression of collagen I (Figure 5H). In all cases, the empty plasmid as a negative control for BACE1 overexpression did not produce any significant changes to the cardiac fibroblasts.
Discussion
Our previous results showed that choline suppresses cardiac fibroblast proliferation and collagen secretion via activating M3 receptor, and this effect can be ascribed to the inhibition of the TGF-β1/Smad and p38 MAPK pathways. However, its specific molecular mechanism has not been elucidated, and the present work was designed to shed light on it.
Key findings
The findings of the present study revealed a novel mechanism by which choline produces the anti-fibrotic action through activating the M3 receptor to upregulate miR-29b, thereby repressing the expression of the downstream target gene BACE1: choline → M3 receptor activation↑ → miR-29b upregulation↑ → BACE1 downregulation↓ → collagen I↓ → fibrosis inhibition↓.
Strengths and limitations
In this study, we proposed for the first time that the activation of M3 receptor can upregulate the expression of miR-29b to exert anti-fibrotic effect, and we identified a target, BACE1, that has never been reported in myocardial fibrosis, which supplemented the mechanism of the anti-fibrotic effects of M3 receptor and further provided new evidence for the function of M3 receptor in myocardial fibrosis.
However, there are some limitations in the present study. For example, how the M3 receptor upregulates miR-29b expression is unclear. It is reported that TGF-β-Smad3-miR-29 isoforms may mainly contribute to the progression of cardiac fibrosis due to Cyclosporine A exposure (25). Previous studies confirmed that the activation of M3 receptor by choline can inhibit the TGF-β/Smad signaling pathway, so we speculated that the fibrotic signaling pathway TGF-β/Smad may act as a bridge between M3 receptor and miR-29b. This still needs to be confirmed by further experiments.
Furthermore, BACE1 was involved in the anti-fibrotic effects of increasing the expression of miR-29b caused by the activation of M3 receptor, and overexpression of BACE1 eliminated the role of miR-29b mimics in cardiac fibrosis. However, it is still unclear whether BACE1 can directly affect the level of collagen I, or whether we can directly act on BACE1 to exert anti-fibrotic effects. This problem is also one of the limitations of this article. We plan to further explore the relationship between BACE1 and cardiac fibrosis in future experiments. Hopefully, it will provide a new strategy for the treatment of cardiac fibrosis.
Comparison with similar researches
In previous studies, we found that M3 receptor are not only expressed in cardiomyocytes, but also in cardiac fibroblasts, and exert anti-fibrotic effects when activated by choline. In this study, we further confirmed that the role of M3 receptor in myocardial fibrosis. But on top of that, we discovered a new member involved in this process, which is miR-29b. Activation of the M3 receptor by choline could promote the expression of miR-29b, and we also found another member, BACE1, a new target of miR-29b in myocardial fibrosis. These results complement our previous research and provide new insights.
Explanations of findings
MiRNAs regulate a variety of pathological processes and have become a research hotspot in the prevention and treatment of diseases in recent years. We screened the miRNAs related to fibrosis and selected miR-29b as the research object to explore whether miR-29b was involved in the anti-fibrotic effect produced by M3 receptor activation. We found that increased miR-29b expression exerted an anti-fibrotic effect in cardiac fibroblasts with exposure to TGF-β1, a master fibrosis inducer, whereas inhibition of miR-29b antagonized the anti-fibrotic effect of M3 receptor. We identified BACE1 as a new target gene for miR-29b in cardiac fibroblasts. The results showed that choline downregulated the protein level of BACE1 by altering the expression of miR-29b, whereas 4-DAMP upregulated its protein level, suggesting that BACE1 was a downstream component of the M3 receptor signaling connecting to cardiac fibrosis. These findings delineated a new molecular/signaling mechanism underlying the protective actions of the M3 receptor and a new mechanism for the development of cardiac fibrosis with miR-29b/BACE1 as new mediators for fibrogenesis.
Previous reports suggested that the M3 receptor is expressed in cardiomyocytes and that the activation of the M3 receptor had a protective effect on arrhythmia (12), myocardial hypertrophy (13), and sudden cardiac death (23). We demonstrated that the M3 receptor level in cardiac fibroblasts was as high as in cardiomyocytes and M3 receptor activation by choline suppressed cardiac fibrosis in mice by inhibiting the P38 MAPK pathway (14). Choline is a precursor for the synthesis of acetylcholine, a non-specific agonist of the M3 receptor. Since 4-DAMP is a specific antagonist of M3 receptor subtype and it counteracts the effect of choline, the effects of choline observed in our experiments can be attributed to M3 receptor activation. However, pharmacological studies suggest that a high-choline diet aggravates cardiac dysfunction, inflammation, and fibrosis in a mouse model of heart failure (26). We consider that such different effects of choline are related to the concentration under study. As our previous results, the anti-fibrotic effect of choline decreased while its concentration increased, suggesting that higher concentrations of choline may bring about the opposite effect, which deserves further investigation.
In this study, we found that miR-29b negatively regulated BACE1 in cardiac fibroblasts and might mediate the anti-fibrotic effect of M3 receptor. BACE1 is a candidate biomarker for Alzheimer’s disease (27). It has been reported that the expression of BACE1 is elevated in both patients and mice with ischemic heart failure and promotes the expression of its antisense transcript BACE1-AS to increase the endothelial cell apoptosis (28). BACE1 can also play a role in ischemic heart disease (29). Three types of myocardial fibrosis have been identified: replacement fibrosis from tissue necrosis, reactive fibrosis from myocardial stress, and infiltrative interstitial fibrosis from progressive deposition of non-degradable material such as amyloid (30). This provides a conjecture for the role of BACE1 in myocardial fibrosis. Our experimental results in this article showed that the expression of BACE1 was increased in cardiac fibroblasts with TGF-β1, which was reversed after transfection with miR-29b mimics. Some studies have reported the targeting relationship between miR-29b and BACE1 in Alzheimer’s disease, thus, in the present study we test the relationship in myocardial fibrosis by western blot rather than dual-luciferase reporter assay. We identified the involvement of the miR-29b/BACE1 axis in the anti-fibrotic effect of the activation of M3 receptor, which is a new molecular mechanism of the activation of M3 receptor to inhibit myocardial fibrosis. However, the role of BACE1 in cardiac fibrosis remains to be further investigated. ST β-galactoside alpha-2,6-sialyltransferase 1 (ST6GAL1) is a type II membrane protein that is commonly localized in the Golgi apparatus, and has been shown to regulate matrix metalloproteinases (MMPs) and vascular endothelial growth factor (VEGF) in lung cancer (31). BACE1 can cleave and release ST6GAL1 from the trans-Golgi into the secretory pathway to regulate the secretion of inflammatory factors in chronic obstructive pulmonary disease (32,33). Moreover, we reviewed the literature and found that NKX-COUP-TFII composite elements (NCCE) in regulatory regions of Madcam1 and St6gal1 are involved in morphogenesis of the heart (34). Meprin β contributes to collagen deposition in lung fibrosis (35), and meprin β complements the role of BACE1 in Alzheimer’s disease (36). These have the potential to play a role in the heart, but follow-up experiments are needed to confirm this.
Implications and actions needed
The experimental results in this study, as well as previous studies of M3 receptors in the heart, suggest that overexpression of M3 receptors may rescue a number of cardiac diseases, such as myocardial hypertrophy, ischemic heart disease, and myocardial fibrosis. In clinical trials, it is also possible to try to improve the level of M3 receptor to treat heart diseases. However, myocardial fibrosis is usually a progressive disease accompanied by other diseases, to achieve the therapeutic effect of overexpression of M3 receptor, it is necessary to take into account the effect of overexpression M3 receptor on other diseases. And there are multiple subtypes of M muscarinic receptors, which also need to be considered when performing therapeutic administration.
The ultimate goal of studying miRNAs is to translate them into clinical applications. MiRNAs can be stabilized in serum and plasma of humans and animals (37). Thus, serum miRNAs can be used as markers for disease diagnosis. In our study, changes of miR-29b in human serum might serve as a marker of myocardial fibrosis disease from progression to remission. In recent years, RNA interference (RNAi) therapeutic technology has emerged based on the study of miRNAs, and some studies have shown that plants can be carriers of certain therapeutic miRNAs (38). MiR-29b could likewise be further investigated in myocardial fibrosis by drawing on these studies. These are all elements that deserve to be explored further.
Conclusions
This study revealed the mechanism by which choline produces the anti-fibrotic action through activating the M3 receptor to upregulate miR-29b, thereby inhibiting the expression of the downstream target gene BACE1.
Acknowledgments
Funding: This work was supported by the National Natural Science Foundation of China (Nos. 81673424, 82073838 and 82273917).
Footnote
Reporting Checklist: The authors have completed the ARRIVE reporting checklist. Available at https://cdt.amegroups.com/article/view/10.21037/cdt-23-309/rc
Data Sharing Statement: Available at https://cdt.amegroups.com/article/view/10.21037/cdt-23-309/dss
Peer Review File: Available at https://cdt.amegroups.com/article/view/10.21037/cdt-23-309/prf
Conflicts of Interest: All authors have completed the ICMJE uniform disclosure form (available at https://cdt.amegroups.com/article/view/10.21037/cdt-23-309/coif). All authors report that this work was supported by National Natural Science Foundation of China. The authors have no other conflicts of interest to declare.
Ethical Statement: The authors are accountable for all aspects of the work in ensuring that questions related to the accuracy or integrity of any part of the work are appropriately investigated and resolved. The study was reviewed and approved by the Ethics Committee of the Second Affiliated Hospital of Harbin Medical University (Approval number: Ky2016-103. Approval time: 9 March 2016), in compliance with the “Laboratory animal—Guideline for ethical review of animal welfare” (GB/T 35892-2018) national guidelines for the care and use of animals.
Open Access Statement: This is an Open Access article distributed in accordance with the Creative Commons Attribution-NonCommercial-NoDerivs 4.0 International License (CC BY-NC-ND 4.0), which permits the non-commercial replication and distribution of the article with the strict proviso that no changes or edits are made and the original work is properly cited (including links to both the formal publication through the relevant DOI and the license). See: https://creativecommons.org/licenses/by-nc-nd/4.0/.
References
- Townsend N, Kazakiewicz D, Lucy Wright F, et al. Epidemiology of cardiovascular disease in Europe. Nat Rev Cardiol 2022;19:133-43. [Crossref] [PubMed]
- Nguyen MN, Kiriazis H, Gao XM, et al. Cardiac Fibrosis and Arrhythmogenesis. Compr Physiol 2017;7:1009-49. [Crossref] [PubMed]
- Ko T, Nomura S, Yamada S, et al. Cardiac fibroblasts regulate the development of heart failure via Htra3-TGF-β-IGFBP7 axis. Nat Commun 2022;13:3275. [Crossref] [PubMed]
- Vähätalo JH, Huikuri HV, Holmström LTA, et al. Association of Silent Myocardial Infarction and Sudden Cardiac Death. JAMA Cardiol 2019;4:796-802. [Crossref] [PubMed]
- Frangogiannis NG. Cardiac fibrosis: Cell biological mechanisms, molecular pathways and therapeutic opportunities. Mol Aspects Med 2019;65:70-99. [Crossref] [PubMed]
- Qiu Y, Song X, Liu Y, et al. Application of recombinant TGF-β1 inhibitory peptide to alleviate isoproterenol-induced cardiac fibrosis. Appl Microbiol Biotechnol 2023;107:6251-62. [Crossref] [PubMed]
- Yang N, Zou C, Luo W, et al. Sclareol attenuates angiotensin II-induced cardiac remodeling and inflammation via inhibiting MAPK signaling. Phytother Res 2023;37:578-91. [Crossref] [PubMed]
- Liu C, Zhou D, Zhang Q, et al. Transcription factor EB (TFEB) improves ventricular remodeling after myocardial infarction by inhibiting Wnt/β-catenin signaling pathway. PeerJ 2023;11:e15841. [Crossref] [PubMed]
- Akhtar H, Al Sudani H, Hussein M, et al. Effects of Renin-Angiotensin-Aldosterone System Inhibition on Left Ventricular Hypertrophy, Diastolic Function, and Functional Status in Patients With Hypertrophic Cardiomyopathy: A Systematic Review. Cureus 2022;14:e26642. [Crossref] [PubMed]
- Saternos HC, Almarghalani DA, Gibson HM, et al. Distribution and function of the muscarinic receptor subtypes in the cardiovascular system. Physiol Genomics 2018;50:1-9. [Crossref] [PubMed]
- Zhao J, Su Y, Zhang Y, et al. Activation of cardiac muscarinic M3 receptors induces delayed cardioprotection by preserving phosphorylated connexin43 and up-regulating cyclooxygenase-2 expression. Br J Pharmacol 2010;159:1217-25. [Crossref] [PubMed]
- Wang S, Han HM, Jiang YN, et al. Activation of cardiac M3 muscarinic acetylcholine receptors has cardioprotective effects against ischaemia-induced arrhythmias. Clin Exp Pharmacol Physiol 2012;39:343-9. [Crossref] [PubMed]
- Wang S, Han HM, Pan ZW, et al. Choline inhibits angiotensin II-induced cardiac hypertrophy by intracellular calcium signal and p38 MAPK pathway. Naunyn Schmiedebergs Arch Pharmacol 2012;385:823-31. [Crossref] [PubMed]
- Zhao L, Chen T, Hang P, et al. Choline Attenuates Cardiac Fibrosis by Inhibiting p38MAPK Signaling Possibly by Acting on M(3) Muscarinic Acetylcholine Receptor. Front Pharmacol 2019;10:1386. [Crossref] [PubMed]
- Bartel DP. MicroRNAs: genomics, biogenesis, mechanism, and function. Cell 2004;116:281-97. [Crossref] [PubMed]
- Amirian M, Jafari-Nozad AM, Darroudi M, et al. Overview of the miR-29 family members' function in breast cancer. Int J Biol Macromol 2023;230:123280. [Crossref] [PubMed]
- Liu MN, Luo G, Gao WJ, et al. miR-29 family: A potential therapeutic target for cardiovascular disease. Pharmacol Res 2021;166:105510. [Crossref] [PubMed]
- Ai K, Yi L, Wang Y, et al. CircRNA_33702 Promotes Renal Fibrosis by Targeting the miR-29b-3p/WNT1-Inducible Signaling Pathway Protein 1 Pathway. J Pharmacol Exp Ther 2023;384:61-71. [Crossref] [PubMed]
- Kong H, Song Q, Hu W, et al. MicroRNA-29a-3p prevents Schistosoma japonicum-induced liver fibrosis by targeting Roundabout homolog 1 in hepatic stellate cells. Parasit Vectors 2023;16:184. [Crossref] [PubMed]
- Li P, Hao X, Liu J, et al. miR-29a-3p Regulates Autophagy by Targeting Akt3-Mediated mTOR in SiO(2)-Induced Lung Fibrosis. Int J Mol Sci 2023;24:11440. [Crossref] [PubMed]
- Yuan J, Yang H, Liu C, et al. Microneedle Patch Loaded with Exosomes Containing MicroRNA-29b Prevents Cardiac Fibrosis after Myocardial Infarction. Adv Healthc Mater 2023;12:e2202959. [Crossref] [PubMed]
- Pan Z, Guo Y, Qi H, et al. M3 subtype of muscarinic acetylcholine receptor promotes cardioprotection via the suppression of miR-376b-5p. PLoS One 2012;7:e32571. [Crossref] [PubMed]
- Liu Y, Sun L, Pan Z, et al. Overexpression of M3 muscarinic receptor is a novel strategy for preventing sudden cardiac death in transgenic mice. Mol Med 2011;17:1179-87. [Crossref] [PubMed]
- Wang C, Wang Y, Fu Z, et al. MiR-29b-3p Inhibits Migration and Invasion of Papillary Thyroid Carcinoma by Downregulating COL1A1 and COL5A1. Front Oncol 2022;12:837581. [Crossref] [PubMed]
- Nourmohammadi K, Bayrami A, Naderi R, et al. Cyclosporine A induces cardiac remodeling through TGF-β/Smad3/miR-29 signaling pathway and alters gene expression of miR-30b-5p/CaMKIIδ isoforms pathways: alleviating effects of moderate exercise. Mol Biol Rep 2023;50:5859-70. [Crossref] [PubMed]
- Shuai W, Wen J, Li X, et al. High-Choline Diet Exacerbates Cardiac Dysfunction, Fibrosis, and Inflammation in a Mouse Model of Heart Failure With Preserved Ejection Fraction. J Card Fail 2020;26:694-702. [Crossref] [PubMed]
- Cervellati C, Vergallo A, Trentini A, et al. Age, Sex, Hypertension and HDL-C Alter Serum BACE1 Activity in Cognitively Normal Subjects: Implications for Alzheimer's Disease. J Prev Alzheimers Dis 2022;9:708-14. [Crossref] [PubMed]
- Greco S, Zaccagnini G, Fuschi P, et al. Increased BACE1-AS long noncoding RNA and β-amyloid levels in heart failure. Cardiovasc Res 2017;113:453-63. [Crossref] [PubMed]
- Gagno G, Ferro F, Fluca AL, et al. From Brain to Heart: Possible Role of Amyloid-β in Ischemic Heart Disease and Ischemia-Reperfusion Injury. Int J Mol Sci 2020;21:9655. [Crossref] [PubMed]
- Karur GR, Aneja A, Stojanovska J, et al. Imaging of Cardiac Fibrosis: An Update, From the AJR Special Series on Imaging of Fibrosis. AJR Am J Roentgenol 2023; Epub ahead of print. [Crossref] [PubMed]
- Yuan Q, Chen X, Han Y, et al. Modification of α2,6-sialylation mediates the invasiveness and tumorigenicity of non-small cell lung cancer cells in vitro and in vivo via Notch1/Hes1/MMPs pathway. Int J Cancer 2018;143:2319-30. [Crossref] [PubMed]
- Kitazume S, Oka R, Ogawa K, et al. Molecular insights into beta-galactoside alpha2,6-sialyltransferase secretion in vivo. Glycobiology 2009;19:479-87. [Crossref] [PubMed]
- Krick S, Helton ES, Easter M, et al. ST6GAL1 and α2-6 Sialylation Regulates IL-6 Expression and Secretion in Chronic Obstructive Pulmonary Disease. Front Immunol 2021;12:693149. [Crossref] [PubMed]
- Dinh TT, Xiang M, Rajaraman A, et al. An NKX-COUP-TFII morphogenetic code directs mucosal endothelial addressin expression. Nat Commun 2022;13:7448. [Crossref] [PubMed]
- Biasin V, Wygrecka M, Marsh LM, et al. Meprin β contributes to collagen deposition in lung fibrosis. Sci Rep 2017;7:39969. [Crossref] [PubMed]
- Marengo L, Armbrust F, Schoenherr C, et al. Meprin β knockout reduces brain Aβ levels and rescues learning and memory impairments in the APP/lon mouse model for Alzheimer's disease. Cell Mol Life Sci 2022;79:168. [Crossref] [PubMed]
- Chen X, Ba Y, Ma L, et al. Characterization of microRNAs in serum: a novel class of biomarkers for diagnosis of cancer and other diseases. Cell Res 2008;18:997-1006. [Crossref] [PubMed]
- Zhang S, Sang X, Hou D, et al. Plant-derived RNAi therapeutics: A strategic inhibitor of HBsAg. Biomaterials 2019;210:83-93. [Crossref] [PubMed]