3D printing from cardiovascular CT: a practical guide and review
Introduction
With the increasing capacity and sophistication of cardiovascular imaging techniques, so too have the demands and needs of clinicians expanded. Echocardiography, computed tomography (CT), magnetic resonance imaging (MRI) and even nuclear medicine images may be presented as three-dimensional (3D) images which demonstrate the spatial relationship of anatomy or pathology in an immediately interpretable fashion. A step further from the mere two-dimensional visualisation of 3D data is the creation of a replica physical 3D model from imaging data. The method to facilitate this transformation is now readily available in the form of 3D printing, sometimes called rapid prototyping. This technology has expanded rapidly over the preceding decades and is now both ubiquitous and accessible to laypeople and clinicians alike. 3D printing has been utilised in the setting of a multitude of cardiovascular diseases and pathologies in recent years and has been used to demonstrate normal cardiovascular anatomy for teaching and research purposes. Because of its volumetric, high resolution images and availability, cardiovascular CT is particularly suited to the requirements of 3D model generation. This review summarises applications, limitations and practical steps required to create a 3D printed model from cardiovascular CT.
Imaging data for 3D printing: general concerns
Essentially any 3D image acquisition may be used to form the basis of a mesh or model which may be 3D printed. The requirements of the data are that each slice or data element acquired must relate to every other image data point with a fixed relationship. The data most suited for 3D printing are isotropic or near isotropic data sets, for example from CT or MRI volume acquisitions, where multiple slices of data exist and in which each slice is contiguous with the preceding slice. Non-isotropic data contain voxels which are not uniform in all three dimensions but may be oblong or elongated in a particular axis. Such non-isotropic datasets can be used, although the underlying model will have poorer resolution in the undersampled dimension.
CT
Of all imaging modalities, CT is generally the easiest modality with which to create a model for 3D printing. The scan parameters used to generate a 3D printed model of a structure will be the same as those that allow the ideal visualisation of the anatomical structure. For example, the contrast timing for a CT pulmonary angiogram will allow the easy delineation of the pulmonary artery and the exclusion of venous structures where 3D printing of the pulmonary artery is desired. A CT coronary angiogram requires contrast to be delivered to the coronary arteries, but the bolus timing should also allow for opacification of the right ventricle if delineation between the right ventricular cavity and interventricular septum is required.
Of key importance to 3D printing is image quality. All artefacts that occur within the image, such as streak artefacts, blooming or beam hardening, will be represented in the 3D mesh which is generated and will also be manifest in the 3D printed object. Motion, such as coronary artery motion, may cause the coronary arteries to be much larger in the 3D printed object than in the real heart unless specific steps are taken to compensate for this issue. Iterative reconstruction (1,2) and more sophisticated de-noising techniques such as four-dimensional (4D) noise reduction (3,4) can be helpful, particularly in paediatric or low-dose imaging (Figure 1).
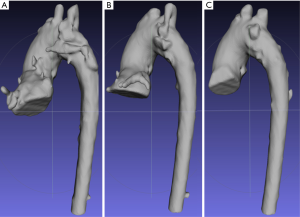
Image voxel size and reconstruction kernels should be selected according to model requirements. Small voxel size and sharp kernels are appropriate for capturing fine image detail but due to noise may require more manual segmentation and post-processing to smooth the model. Conversely, larger voxel size and ‘soft’ reconstruction kernels may facilitate automatic segmentation (5) and produce smoother 3D models at the cost of loss of model detail.
Careful attention to scan parameters may assist in the process of mesh generation and create higher quality models. With regards to scan acquisition settings, where high quality 3D printing is required, it is better to err on the side of higher mA (or kV depending on body habitus) to ensure noise is minimised. Low kV CT imaging can be helpful for accentuating vessel and blood pool contrast for a given injected contrast volume. Likewise, contrast administration should be sufficient to allow clear delineation between the required anatomy and extraneous structures. Two or three phase contrast dosing (for example using a 50:50 contrast mix after the main contrast bolus with further saline chaser) can be useful to delineate the interventricular septum from the right ventricular cavity for myocardial printing and this strategy is also helpful for capturing atrial septal defects. In contradistinction, full strength contrast mixing with the unpacified blood from the inferior vena cava can make delineation of right atrial structures difficult.
Lastly, with regards to ECG gating, ungated imaging leads to motion artefact and blurring of structures’ edge definition. This can translate to an overestimate of the size of objects in the 3D printed model or the inadvertent merging of anatomical structures. While non-gated images can be used to represent gross anatomy, gating is recommended for producing cardiovascular 3D models where exact dimensions are necessary and it is obligatory where small structures such as the coronary arteries are to be reproduced.
It is important to underline certain forms of image data which are not suitable for printing. Maximum intensity projection (MIP), thick slabs and static images of a 3D computationally derived objects such as a volume rendered image are not suitable for 3D printing. Volume rendering in general is not helpful for 3D printing, as the process of volume rendering applies transparency to different objects contingent on the underlying Hounsfield unit, while transparency is not generally achievable within 3D printing. By contrast, surface-rendered images approximate a 3D print. The surface contour sharply defines the object as present or absent, typically through the use of Hounsfield unit isocontours, and this binary state can be converted to a mesh for 3D printing. The difference between volume-rendered and surface-rendered images is illustrated in Figure 2.
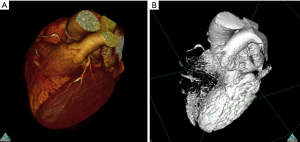
Mesh generation
After 3D imaging data has been acquired, the next step is to create a mesh (Figures 3-6), which is a representation of the outer surface of the object to be 3D printed. A mesh is generated by dividing these images into regions based on properties such as brightness or contrast through a process known as segmentation. A number of medical image segmentation software programs are available and have been reviewed previously (6,7). Some are open-source and freely accessible, while others are commercial, licenced products.


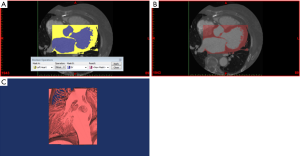
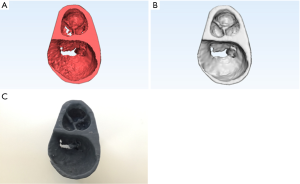
Segmentation may involve manually drawing contours or different thresholds on each image or drawing contours or thresholds on non-contiguous images and interpolating between these contours, which can then be smoothed to form a 3D object (8). More usefully, there are a number of automated techniques to generate a surface for 3D printing. These can include segmentation based on threshold measurements, region growing techniques and the inclusion or exclusion of selected image elements.
In general, models derived from the contrast enhanced blood pool are most robust and direct segmentation of soft tissues is more difficult. A common technique used to produce a model of tissue surrounding a ventricular cavity or blood vessel is to create a model of the blood pool and then create a shell surrounding the vessel/cavity model (Figure 7). The shell can be adjusted to a desired thickness. A representation rather than exact replica of vascular or myocardial tissue is created, but this technique appears more robust, at least in certain clinical settings (9).
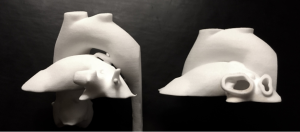
Multiple different file formats for 3D meshes exist. One of the oldest and most widespread is the STL or stereolithography format, which has been used for more than two decades. This breaks down a 3D object into a series of vertices of triangles of various sizes (Figure 8). While simple, this format has a number of deficiencies and it is not uncommon to generate an STL file which contains errors or is otherwise unprintable due to small holes or defects, as well as other errors such as ‘inverted triangles’ or ‘bad edges’. STL files may require repairing prior to printing contingent on available printing software. STL files do not contain colour information and in order to print in multiple colours, a different file format will need to be used. Other file formats (such as the VRML format) store the geometrical mesh along with a colour map. This colour map is laid over the 3D model and is interpreted by the 3D printer to enable multiple different parts to be coloured in different ways. A multitude of other file formats exist and different file formats may be required by different forms of 3D printers.
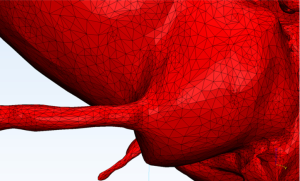
Methods of 3D printing
Multiple kinds of 3D printers exist which use different technology to produce 3D printed objects. Examples of different printer outputs are illustrated in Figure 9. 3D printers have varying properties and range in price over several orders of magnitude. An understanding of the advantages and limitations of each form of 3D printer is helpful and ideal selection is contingent on the application required.
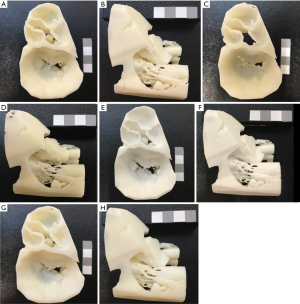
Material extrusion
Material extrusion, also known as fused fibre filament (FFF) or fused deposition modelling (FDM) printers, are the most common types of printers that use material extrusion technology. They create a 3D model by passing a wire of plastic into a heated extruder, which is a nozzle that releases the thin strip of plastic while moving across a print bed (Figure 10). In this way, a 3D model is built up layer by layer by laying down thin strips of plastic material. As each layer is printed, either the print bed or the nozzle moves to allow a second layer to be built upon the first. This method of printing is widely available and printers may be bought for under one thousand US dollars. The material used is generally low cost and readily available. The print quality is dependent on the exact printer but can be quite high, with resolutions in the order of 0.2 mm. A glossy finish may be achieved by treating the model (if ABS - acrylonitrile butadiene styrene - plastic is used) with acetone. A variety of print materials such as PLA and composite materials are available for printing. A major limitation of this 3D printing technique is the ability to deal with overhangs; that is, roofs of material which are not inherently supported by any underlying structure. An example would be the left ventricular cavity. A material extrusion printer may have difficulty printing the top of the ventricular cavity, as there is no underlying structure to support each layer of plastic as it is laid down. To get around this issue, supports are frequently printed (Figure 11). These are small columns of material which hold up overlying structures and allow the 3D printer to build layers in areas which would otherwise be unsupported. These columns may be broken off and removed to produce the final print. Some printers have a second extrusion nozzle which lays down soluble support material. The soluble supports can be dissolved with water or other solvents to achieve the final 3D print.
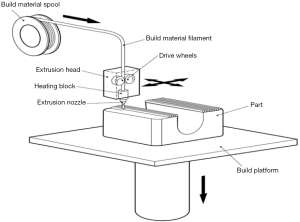
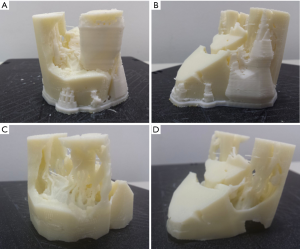
Vat polymerisation
Vat polymerisation or stereolithography (SLA) printers use a directed light source to excite a photopolymer. Using a projector or laser, each layer of material is polymerised and attached to a print bed to build up multiple layers (Figure 12). As with material extrusion printers, there can be some difficulties with unsupported structures, although as a liquid bath is used during construction this may be less problematic. Vat polymerisation printers may also produce very fine structures. Vat polymerisation printers are used in the jewellery industry, but may be also useful for printing coronary arteries or valve tissue which requires fine and accurate structures to be formed. Vat polymerisation printers are modestly priced and easily available. Resin costs are higher than the plastic used in material extrusion printers and there may also be certain stickiness or residue immediately after printing, requiring further processing.
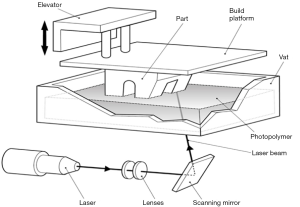
Powder bed fusion
Selective laser sintering (SLS) printers are the protypic printers that use powder bed fusion technology (8,10). Powder bed fusion printers use a laser to heat and fuse a bed of powdered printing material such as nylon (Figure 13). The entire bed is heated up close to the melting point of the nylon powder and the laser melts and binds each layer of the 3D print. After a layer is printed, rollers place a small layer of powder above the printed layer which is then fused to join to the underlying layer of material. In this manner, multiple layers are combined to form a 3D printed object. Nylon is often used as a low cost, robust material that may be sterilisable. Other materials including titanium have been used for printing prostheses such as bone implants (11). This printing method has the advantage of not requiring any support structures and the printed part is generally robust. The printers required for this are generally quite expensive at the current time, although the material costs are low when prints take place in large batches.

Binder jetting
Printers that use binder jetting technology use a binder, such as glue, to adhere layers of a fine material, such as finely ground plaster or plastic particulates together layer by layer (Figure 14). After one layer of the print has been bound, another layer of powder is added to the bed until a 3D model is printed. The majority of multi-colour printers use a binder jetting technique. The outer layer or visible portion of each slice in the model can be coloured in a similar method to ink jet printing after the binding material has been jetted onto the powder. These models may be visually attractive and can be used to represent different anatomical structures. Binder jetting printers are generally modestly expensive and printed parts all need to be vacuumed or cleaned at the time of printing, typically in a dedicated work station to avoid dispersal of fine dust particles. Depending on the precise technology, these models can be very fragile and may require post-processing, such as impregnation with cyanoacrylate (‘superglue’), to improve the stability of the parts.

Material jetting
The final common method of printing is material jetting. This involves multiple small jets laying down a photopolymer above an underlying layer (Figure 15). These jets may lay down various forms of plastic or rubber-like materials in multiple colours. It may also lay down a support material. Each layer is exposed to UV light which causes the liquid material to harden. Using this method, multiple layers are built up to form the 3D model. Soluble material which may be meltable wax or water soluble photopolymer are removed to present the final object. These printers are generally expensive, but may allow the printing of an object with multiple material types. The material properties may also be altered, for example to add flexibility to certain areas of the anatomy. While material characteristics can vary, currently available materials cannot replicate the full range of biological tissue characteristics.
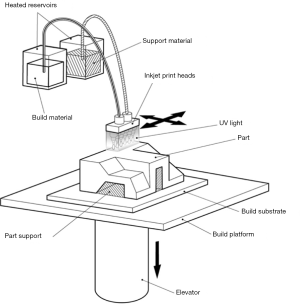
Applications
Given recent advances that have occurred in medical imaging, digital reconstruction and 3D printing, this technology has shown widespread applications in both educational and clinical settings, which have been previously described in the literature (12-17).
Education
3D printing has been recognised as a powerful tool for medical education. Anatomical specimens, as well as negative spaces such as sinuses and blood vessels, have been digitised and 3D printed to form highly realistic and accurate replicas (18-21). These replicas can be used for teaching and remove the expense, hazards and difficulties associated with formalin-based cadaveric specimens. They also offer the advantage of durability compared to plastinated models. Such replicas have been used successfully in anatomy education, with previous studies showing that this may benefit students and improve academic results (19), and that students have positive perceptions towards these resources (18). To enhance the effectiveness of these models, a synthetic colour may be used to highlight various anatomical features. Heart and other anatomical specimens have been produced in this way (19,20).
Physicians and patients alike may also benefit from the use of 3D printed models. 3D printing has been utilised to produce cardiovascular models for surgical training purposes (18,22). Through simulation training using these models, the skills of surgeons can be improved and their confidence increased (13,14). The use of 3D printed training models for this purpose could reduce the risk of surgical complications during the early learning curve period and reduce the need for animal models. The illustration of pathologies, surgical procedures and treatment options for patients may also be facilitated through the use of 3D printed models, enhancing patient-clinician interactions, such as obtaining informed consent (23,24). For example, in a randomised controlled trial (25), a standard teaching session using 3D printed models for paediatric resident education was more highly rated than a session without the models. Learner satisfaction scores were also higher in this session, which correlated with subject matter retention in adult learners. Image and 3D file repositories of cardiovascular pathologies are available to aid further education in congenital heart disease (26).
In an educational setting, the cost and limited durability of some 3D printed models may restrict their availability for teaching purposes in universities and other education facilities. Currently available 3D printers are also limited by their dimensions, meaning that it may not be possible to replicate large anatomical specimens unless the model is re-scaled or assembled using multiple parts. Additionally, while 3D printing materials display varying properties, they do not mimic the true nature of real human tissues as in cadaveric specimens, although this may not impact educational outcomes (19).
Surgical planning
A major clinical application of 3D printing to date has been in surgical planning, particularly for the repair of congenital heart defects. Congenital surgery is difficult, requiring a thorough understanding of patient anatomy which can often be complex, variable and unpredictable. A tangible representation of these complex anatomic structures may therefore be helpful to pre-prepare a surgeon for a variety of treatment options and enhance communication when members of different disciplines will participate in a procedure. Various studies have demonstrated the benefits of using physical 3D models for detailed morphological analysis and to facilitate intraoperative orientation in cases of complex congenital pathologies (Table 1). In some instances, patches and other materials have also been pre-formed on the basis of 3D printed data. Additionally, virtual models produced from MRI data have proven useful in simulating surgical interventions and planning for congenital defect repair (39-41).
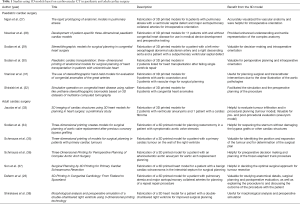
Full table
Aside from planning of congenital repair procedures, most other cardiac applications of 3D printing have been to model cardiac tumours in preparation for resection, as well as the aorta to plan for complex replacement surgeries (35-37). In these instances, as well as in the case of congenital heart defects, the use of physical 3D models has proven useful for determining the most appropriate surgical approach in order to avoid procedural complications and increase the likelihood of success (Figures 16,17).
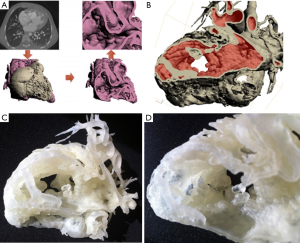
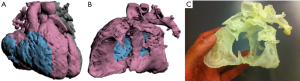
Interventional cardiology
3D printing has potentially valuable applications in the field of interventional cardiology, which involves the use of catheter-based procedures (17,42). In order for these minimally invasive techniques to be applied successfully, a comprehensive knowledge of patient-specific anatomy is crucial. Tangible models may provide proceduralist with a greater appreciation of 3D cardiovascular anatomy, which can be useful in planning the treatment of high-risk patients for which conventional surgery is often deemed unsuitable.
Given that tissue characteristics can be replicated in 3D models by using flexible materials, it is possible to simulate interventions to determine procedure feasibility and predict potential outcomes. Limited studies have shown that in procedures such as valve repair or replacement (34,43-45), as well as left atrial appendage, septal defect and aortic pseudoaneurysm closures (46-50), the use of 3D printed models has facilitated appropriate device selection and post-procedural evaluation of device deployment (Table 2).
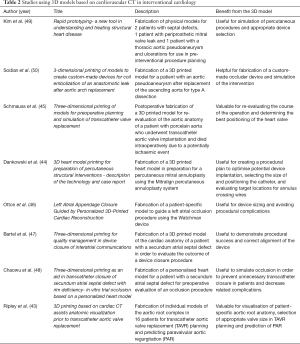
Full table
Cardiovascular 3D printing in clinical practice
While 3D printing is intuitively attractive, there are several impediments to widespread implementation. The process of mesh generation can be partly automated but for clinical use, great care must be taken during this process. The underlying mesh is contingent on the underlying image quality and complex anatomy or congenital abnormalities may be difficult to correctly interpret and classify. Careful judgement is often required when setting threshold values and when adjusting manual segmentation contours. Expert involvement in 3D file generation or close supervision is therefore required.
Each model is subject to multiple steps of processing between image acquisition and 3D printing. 3D printing itself may lead to errors or minor (<3%) model shrinkage (51-54). Quality control steps or model validation are therefore required where 3D printed models are used for device sizing. For example, the produced 3D or STL file can be projected back onto the source DICOM data to ensure accuracy. More sophisticated and robust quality control mechanisms involve the re-scanning of the final model with CT or 3D optical scanning techniques and comparing the scanned 3D printed model to the source STL file and DICOM data (Figure 18).
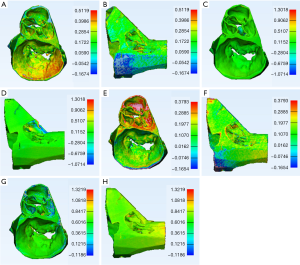
3D printers remain expensive and require ongoing maintenance. Several 3D printer designs are more efficient when batch printing large volumes of models and in such cases, the economics and convenience of bureau 3D printing services can outweigh the benefits of on-site machines. Bureau services, which are widely available, also allow clinicians and researchers to trial different printer techniques and ideally match the printer to study question at hand.
In a clinical setting, the use of physical models is currently only possible in the case of elective or semi-elective procedures. This is because of the time required to produce a model from patient imaging data, with 6-12 hours as a minimum expected for a high quality heart-sized print.
Future directions
3D printers are evolving rapidly and the cost and accuracy of 3D printing continues to improve, as does the versatility and range of available print materials. Generating 3D files such as STL files from DICOM data is being recognised as clinical necessity and this capacity is being introduced into the clinical advanced visualisation software and DICOM viewers offered by many vendors.
While 3D printed patient-specific prostheses have emerged in fields such as orthopaedics, cardiovascular 3D printed implants remain in development. Demonstrating suitability and durability of patient-specific prostheses remains challenging with important regulatory implications (55).
True biological printing, that is the printing of a biocompatible scaffold with stem cells capable of replicating or replacing biological tissue, remains an ambitious future goal of 3D printing. Biological printing has succeeded in establishing thin sheets of tissue that are biologically active and can be used for pharmaceutical testing (56). However, replication of cardiovascular tissue, even ‘simple’ avascular tissue such as a heart valve, is problematic (57) and 3D printing is likely to help overcome only a few of the many impediments to the realisation of this ambition. The role of 3D printing in cardiovascular tissue engineering has been reviewed elsewhere (58).
Conclusions
Cardiovascular 3D printing for both educational and clinical purposes is highly promising and is likely to expand in its use and application. While challenges exist in terms of the economic cost of producing 3D prints, as well as limits on the materials available, these current limitations of 3D printing are likely to be overcome with further research and technological advances.
To date, 3D printing has focussed on broadening and demonstrating the applications of the technology, frequently in case reports and small case series. In order to establish the use of 3D printing within routine clinical practice, future studies will need to focus on demonstrable resource-appropriate clinical outcomes from the use of 3D printing. Given the trajectory of 3D printing technology and its demonstrated utility in multiple clinical scenarios, the widespread application of 3D printing in clinical cardiovascular care seems inevitable.
Acknowledgements
This work was supported by the Surgical and Orthopaedic Research Laboratory, Prince of Wales Clinical School, UNSW Australia; the Michael Crouch Innovation Centre, UNSW Australia; and Objective3D Advanced Manufacturing Centre, Victoria, Australia. Dr James Otton would like to acknowledge research support from South West Sydney Local Health District and grant support from the St Vincent’s Clinic Foundation, Sydney Australia. We would like to acknowledge Lorenzo Lucia for technical illustrations.
Footnote
Conflicts of Interest: The authors have no conflicts of interest to declare.
References
- Lee JW, Lee G, Lee NK, et al. Effectiveness of Adaptive Statistical Iterative Reconstruction for 64-Slice Dual-Energy Computed Tomography Pulmonary Angiography in Patients With a Reduced Iodine Load: Comparison With Standard Computed Tomography Pulmonary Angiography. J Comput Assist Tomogr 2016;40:777-83. [Crossref] [PubMed]
- Ryu YJ, Choi YH, Cheon JE, et al. Knowledge-based iterative model reconstruction: comparative image quality and radiation dose with a pediatric computed tomography phantom. Pediatr Radiol 2016;46:303-15. [Crossref] [PubMed]
- Otton JM, Kühl JT, Kofoed KF, et al. Four-dimensional image processing of myocardial CT perfusion for improved image quality and noise reduction. J Cardiovasc Comput Tomogr 2013;7:110-6. [Crossref] [PubMed]
- Nishii T, Kono AK, Tani W, et al. Four-dimensional noise reduction using the time series of medical computed tomography datasets with short interval times: a static-phantom study. PeerJ 2016;4:e1680. [Crossref] [PubMed]
- Tandon A, Byrne N, Nieves Velasco Forte Mde L, et al. Use of a semi-automated cardiac segmentation tool improves reproducibility and speed of segmentation of contaminated right heart magnetic resonance angiography. Int J Cardiovasc Imaging 2016;32:1273-9. [Crossref] [PubMed]
- Withey DJ, Koles ZJ. A review of medical image segmentation: methods and available software. International Journal of Bioelectromagnetism 2008;10:125-48.
- Byrne N, Velasco Forte M, Tandon A, et al. A systematic review of image segmentation methodology, used in the additive manufacture of patient-specific 3D printed models of the cardiovascular system. JRSM Cardiovasc Dis 2016;5:2048004016645467. [Crossref] [PubMed]
- Greil GF, Kuettner A, Flohr T, et al. High-resolution reconstruction of a waxed heart specimen with flat panel volume computed tomography and rapid prototyping. J Comput Assist Tomogr 2007;31:444-8. [Crossref] [PubMed]
- Farooqi KM, Lengua CG, Weinberg AD, et al. Blood Pool Segmentation Results in Superior Virtual Cardiac Models than Myocardial Segmentation for 3D Printing. Pediatr Cardiol 2016;37:1028-36. [Crossref] [PubMed]
- Greil GF, Wolf I, Kuettner A, et al. Stereolithographic reproduction of complex cardiac morphology based on high spatial resolution imaging. Clin Res Cardiol 2007;96:176-85. [Crossref] [PubMed]
- Park EK, Lim JY, Yun IS, et al. Cranioplasty Enhanced by Three-Dimensional Printing: Custom-Made Three-Dimensional-Printed Titanium Implants for Skull Defects. J Craniofac Surg 2016;27:943-9. [Crossref] [PubMed]
- Schmauss D, Haeberle S, Hagl C, et al. Three-dimensional printing in cardiac surgery and interventional cardiology: a single-centre experience. Eur J Cardiothorac Surg 2015;47:1044-52. [Crossref] [PubMed]
- Shi D, Liu K, Zhang X, et al. Applications of three-dimensional printing technology in the cardiovascular field. Intern Emerg Med 2015;10:769-80. [Crossref] [PubMed]
- Rengier F, Mehndiratta A, von Tengg-Kobligk H, et al. 3D printing based on imaging data: review of medical applications. Int J Comput Assist Radiol Surg 2010;5:335-41. [Crossref] [PubMed]
- Matsumoto JS, Morris JM, Foley TA, et al. Three-dimensional Physical Modeling: Applications and Experience at Mayo Clinic. Radiographics 2015;35:1989-2006. [Crossref] [PubMed]
- Mitsouras D, Liacouras P, Imanzadeh A, et al. Medical 3D Printing for the Radiologist. Radiographics 2015;35:1965-88. [Crossref] [PubMed]
- Gómez-Ciriza G, Hussain T, Gómez-Cía T, et al. Potential of 3D-printed models in planning structural interventional procedures. Interventional Cardiology 2015;7:345-52. [Crossref]
- O'Reilly MK, Reese S, Herlihy T, et al. Fabrication and assessment of 3D printed anatomical models of the lower limb for anatomical teaching and femoral vessel access training in medicine. Anat Sci Educ 2016;9:71-9. [Crossref] [PubMed]
- Lim KH, Loo ZY, Goldie SJ, et al. Use of 3D printed models in medical education: A randomized control trial comparing 3D prints versus cadaveric materials for learning external cardiac anatomy. Anat Sci Educ 2016;9:213-21. [Crossref] [PubMed]
- McMenamin PG, Quayle MR, McHenry CR, et al. The production of anatomical teaching resources using three-dimensional (3D) printing technology. Anat Sci Educ 2014;7:479-86. [Crossref] [PubMed]
- AbouHashem Y, Dayal M, Savanah S, et al. The application of 3D printing in anatomy education. Med Educ Online 2015;20:29847. [Crossref]
- Reuthebuch O, Lang A, Groscurth P, et al. Advanced training model for beating heart coronary artery surgery: the Zurich heart-trainer. Eur J Cardiothorac Surg 2002;22:244-8. [Crossref] [PubMed]
- Deferm S, Meyns B, Vlasselaers D, et al. 3D-Printing in Congenital Cardiology: From Flatland to Spaceland. J Clin Imaging Sci 2016;6:8. [Crossref] [PubMed]
- Biglino G, Capelli C, Wray J, et al. 3D-manufactured patient-specific models of congenital heart defects for communication in clinical practice: feasibility and acceptability. BMJ Open 2015;5:e007165. [Crossref] [PubMed]
- Loke T, Krieger A, Sable C, et al. Novel Uses for Three-Dimensional Printing in Congenital Heart Disease. Current Pediatrics Reports 2016;4:28-34. [Crossref]
- Health NIo. NIH 3D print exchange. 2014. Available online: https://3dprint.nih.gov/
- Ngan EM, Rebeyka IM, Ross DB, et al. The rapid prototyping of anatomic models in pulmonary atresia. J Thorac Cardiovasc Surg 2006;132:264-9. [Crossref] [PubMed]
- Noecker AM, Chen JF, Zhou Q, et al. Development of patient-specific three-dimensional pediatric cardiac models. ASAIO J 2006;52:349-53. [Crossref] [PubMed]
- Sodian R, Weber S, Markert M, et al. Stereolithographic models for surgical planning in congenital heart surgery. Ann Thorac Surg 2007;83:1854-7. [Crossref] [PubMed]
- Sodian R, Weber S, Markert M, et al. Pediatric cardiac transplantation: three-dimensional printing of anatomic models for surgical planning of heart transplantation in patients with univentricular heart. J Thorac Cardiovasc Surg 2008;136:1098-9. [Crossref] [PubMed]
- Vranicar M, Gregory W, Douglas WI, et al. The use of stereolithographic hand held models for evaluation of congenital anomalies of the great arteries. Stud Health Technol Inform 2008;132:538-43. [PubMed]
- Shiraishi I, Yamagishi M, Hamaoka K, et al. Simulative operation on congenital heart disease using rubber-like urethane stereolithographic biomodels based on 3D datasets of multislice computed tomography. Eur J Cardiothorac Surg 2010;37:302-6. [PubMed]
- Jacobs S, Grunert R, Mohr FW, Falk V. 3D-Imaging of cardiac structures using 3D heart models for planning in heart surgery: a preliminary study. Interact Cardiovasc Thorac Surg 2008;7:6-9. [Crossref] [PubMed]
- Sodian R, Schmauss D, Markert M, et al. Three-dimensional printing creates models for surgical planning of aortic valve replacement after previous coronary bypass grafting. Ann Thorac Surg 2008;85:2105-8. [Crossref] [PubMed]
- Schmauss D, Gerber N, Sodian R. Three-dimensional printing of models for surgical planning in patients with primary cardiac tumors. J Thorac Cardiovasc Surg 2013;145:1407-8. [Crossref] [PubMed]
- Schmauss D, Juchem G, Weber S, et al. Three-dimensional printing for perioperative planning of complex aortic arch surgery. Ann Thorac Surg 2014;97:2160-3. [Crossref] [PubMed]
- Son KH, Kim KW, Ahn CB, et al. Surgical Planning by 3D Printing for Primary Cardiac Schwannoma Resection. Yonsei Med J 2015;56:1735-7. [Crossref] [PubMed]
- Shirakawa T, Koyama Y, Mizoguchi H, et al. Morphological analysis and preoperative simulation of a double-chambered right ventricle using 3-dimensional printing technology. Interact Cardiovasc Thorac Surg 2016;22:688-90. [Crossref] [PubMed]
- Sørensen TS, Greil GF, Hansen OK, et al. Surgical simulation--a new tool to evaluate surgical incisions in congenital heart disease? Interact Cardiovasc Thorac Surg 2006;5:536-9. [Crossref] [PubMed]
- Sørensen TS, Mosegaard J, Greil GF, et al. Images in cardiovascular medicine. Virtual cardiotomy for preoperative planning. Circulation 2007;115:e312. [Crossref] [PubMed]
- Sørensen TS, Beerbaum P, Mosegaard J, et al. Virtual cardiotomy based on 3-D MRI for preoperative planning in congenital heart disease. Pediatr Radiol 2008;38:1314-22. [Crossref] [PubMed]
- Vaquerizo B, Theriault-Lauzier P, Piazza N. Percutaneous Transcatheter Mitral Valve Replacement: Patient-specific Three-dimensional Computer-based Heart Model and Prototyping. Rev Esp Cardiol (Engl Ed) 2015;68:1165-73. [Crossref] [PubMed]
- Ripley B, Kelil T, Cheezum MK, et al. 3D printing based on cardiac CT assists anatomic visualization prior to transcatheter aortic valve replacement. J Cardiovasc Comput Tomogr 2016;10:28-36. [Crossref] [PubMed]
- Dankowski R, Baszko A, Sutherland M, et al. 3D heart model printing for preparation of percutaneous structural interventions: description of the technology and case report. Kardiol Pol 2014;72:546-51. [Crossref] [PubMed]
- Schmauss D, Schmitz C, Bigdeli AK, et al. Three-dimensional printing of models for preoperative planning and simulation of transcatheter valve replacement. Ann Thorac Surg 2012;93:e31-3. [Crossref] [PubMed]
- Otton JM, Spina R, Sulas R, et al. Left Atrial Appendage Closure Guided by Personalized 3D-Printed Cardiac Reconstruction. JACC Cardiovasc Interv 2015;8:1004-6. [Crossref] [PubMed]
- Bartel T, Rivard A, Jimenez A, et al. Three-dimensional printing for quality management in device closure of interatrial communications. Eur Heart J Cardiovasc Imaging 2016;17:1069. [Crossref] [PubMed]
- Chaowu Y, Hua L, Xin S. Three-Dimensional Printing as an Aid in Transcatheter Closure of Secundum Atrial Septal Defect With Rim Deficiency: In Vitro Trial Occlusion Based on a Personalized Heart Model. Circulation 2016;133:e608-10. [Crossref] [PubMed]
- Kim MS, Hansgen AR, Wink O, et al. Rapid prototyping: a new tool in understanding and treating structural heart disease. Circulation 2008;117:2388-94. [Crossref] [PubMed]
- Sodian R, Schmauss D, Schmitz C, et al. 3-dimensional printing of models to create custom-made devices for coil embolization of an anastomotic leak after aortic arch replacement. Ann Thorac Surg 2009;88:974-8. [Crossref] [PubMed]
- Ibrahim D, Broilo TL, Heitz C, et al. Dimensional error of selective laser sintering, three-dimensional printing and PolyJet models in the reproduction of mandibular anatomy. J Craniomaxillofac Surg 2009;37:167-73. [Crossref] [PubMed]
- Taft RM, Kondor S, Grant GT. Accuracy of rapid prototype models for head and neck reconstruction. J Prosthet Dent 2011;106:399-408. [Crossref] [PubMed]
- Choi JY, Choi JH, Kim NK, et al. Analysis of errors in medical rapid prototyping models. Int J Oral Maxillofac Surg 2002;31:23-32. [Crossref] [PubMed]
- Hazeveld A, Huddleston Slater JJ, Ren Y. Accuracy and reproducibility of dental replica models reconstructed by different rapid prototyping techniques. Am J Orthod Dentofacial Orthop 2014;145:108-15. [Crossref] [PubMed]
- Hockaday LA, Kang KH, Colangelo NW, et al. Rapid 3D printing of anatomically accurate and mechanically heterogeneous aortic valve hydrogel scaffolds. Biofabrication 2012;4:035005. [Crossref] [PubMed]
- Ma X, Qu X, Zhu W, et al. Deterministically patterned biomimetic human iPSC-derived hepatic model via rapid 3D bioprinting. Proc Natl Acad Sci U S A 2016;113:2206-11. [Crossref] [PubMed]
- Cheung DY, Duan B, Butcher JT. Current progress in tissue engineering of heart valves: multiscale problems, multiscale solutions. Expert Opin Biol Ther 2015;15:1155-72. [Crossref] [PubMed]
- Mosadegh B, Xiong G, Dunham S, et al. Current progress in 3D printing for cardiovascular tissue engineering. Biomed Mater 2015;10:034002. [Crossref] [PubMed]