Hemostasis and nanotechnology
Introduction
Bleeding results from disruption of the vessel wall integrity. It can present in a variety of manifestations, both micro- and macroscopic, affecting all ages and genders. Whether through trauma or as a result of an underlying medical condition, the goal of the clinician is to identify and stop the bleeding.
The pathophysiologic consequences of bleeding can be severe, resulting in metabolic and cellular dysfunction secondary to deficiencies in oxygen delivery. If not stabilized, it can lead to hypoxia, end-organ damage, and death. The maximum duration of ischemia without deleterious effects is variable for each organ and depends heavily on its metabolic requirements. The extent of cellular injury and dysfunction is a multifactorial process dependent on both the degree and duration of ischemia (1). Thus, prompt restoration of blood flow remains the best therapeutic option for treatment. Despite optimal treatment strategies, hemorrhage due to vascular trauma accounts for 40% of all trauma-related deaths (2). In particular, intracerebral hemorrhage mortality ranges from 35% to 52% and gastrointestinal bleeding carries a mortality rate of 5–10% in the United States and United Kingdom (3,4).
Innate hemostasis
The body’s innate response to vascular injury is a dynamic process that involves both physical and chemical interactions to promote platelet aggregation and the formation of a stable clot. A quick and localized response is ideal to control the bleed while minimizing unwanted systemic thrombosis. The hemostatic process can be described in three steps; platelet plug formation, activation of the coagulation cascade, and removal of the clot through fibrinolysis (Figure 1) (5).
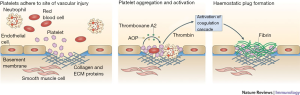
In response to blood vessel trauma, the smooth muscle cells that line vessels contract in order to slow blood flow to the area. This vasoconstriction is crucial in slowing hemorrhage; in small vessels, these spasms can completely halt bleeding, and in larger vessels can significantly restrict blood outflow and reduce damage to the area (6).
Following the initial injury and spasm, platelets aggregate to form a clot. Under normal conditions, platelets do not adhere to the vascular endothelium secondary to antithrombotic nitric oxide and prostacyclin production. However, the inciting injury exposes subendothelium that contains highly thrombogenic molecules such as collagen, von Willebrand factor (vWF), and other procoagulant proteins (7,8). Quiescent platelets first bind the endothelium via vWF. A more stable adhesion is made next after binding collagen (9). Subsequently, platelets become activated through the functions of adenosine diphosphate (ADP), epinephrine, thrombin, and collagen. Once activated, platelets undergo a shape change, further enhancing adhesion to the subendothelium (8). Activated platelets also express the glycoprotein IIb/IIIa receptor which mediates platelet to platelet aggregation. A variety of substances secreted by activated platelets, including thromboxane A2 (TxA2), cause more platelets to influx to the site of the vascular injury, resulting in the formation of a weak hemostatic plug (6).
After a weak platelet plug is formed, the end product of the coagulation cascade, fibrin, works to strengthen and stabilize the clot. Fibrin is converted from fibrinogen through the action of thrombin to form a fibrin scaffold. In addition, an extracellular genomic DNA/histone scaffold within the thrombus matrix provides support and may play a key role in chronic thrombus (10).
The classical view of the coagulation cascade consisting of an intrinsic and extrinsic pathway has been replaced with a more physiologically accurate cascade. The primary inciting event is due to the interaction between tissue factor and activated factor VII at the site of injury. The thrombin generated then activates platelets and cofactors through an amplification feedback mechanism, further forming more fibrin to stabilize the clot (11,12).
With the rapid amplification of clot propagation, anti-thrombotic mechanisms to halt the process become important. Termination involves primarily antithrombin, tissue factor pathway inhibitor (TFPI), and the protein C pathway (13-15).
In the final step, the clot is removed by fibrinolysis. The process begins with plasminogen binding fibrin and tissue plasminogen activator (tPA), which forms active plasmin. Plasmin cleaves fibrin, fibrinogen, and a variety of plasma proteins and clotting factors, dissolving the clot (16). When these processes become altered, it can lead to pathologic clot formation such as venous thromboembolism, contributing to significant morbidity and utilization of healthcare resources (17-19).
Current hemostatic techniques
In cases where platelet plug formation does not provide enough hemostasis, a variety of additional modalities, including mechanical and thermal, can aid in coagulation. Traditional examples include application of pressure, ligatures, and cautery. Topical hemostatic agents have been developed to augment this process. These have designated uses either extra- or intravascularly by providing a substrate to promote hemostasis or by enhancing the innate coagulation system.
Matrix agents
Matrix agents provide a substrate for thrombus formation. Products are comprised of different macromolecules, including cellulose (Surgicel, Fibrillar, SNoW), gelatin (Gelfoam, Surgifoam), polysaccharides (Arista AH), or collagen (Avitene, Helistat, Helitene). They are formulated as sheets, gels, or powders applied directly onto the bleed and are most effective for gentle rather than brisk bleeding. They are easy to use, absorbable, but are not universally effective as they rely on the presence of a functional coagulation system to stimulate platelet aggregation (20).
External agents
External agents are optimal in non-hospital settings, such as combat, when traditional pressure methods are not sufficient and surgical exploration is not immediately available. Many of the efficacy studies come from animal models and robust clinical studies are lacking. Most available clinical data is through case reports or survey-based (21).
Zeolites
Hemostatic agents comprised of zeolites, such as QuikClot, contain granules of inert minerals such as silicon, aluminum, magnesium, and sodium. They rapidly adsorb water and concentrate platelets, erythrocytes and clotting factors when placed on a wound (22,23). QuikClot Combat Gauze is a second-generation product made of non-woven gauze coated with kaolin, an aluminosilicate clay that activates factor XII of the coagulation pathway. It is approved by the United States Food and Drug Administration (FDA) and is currently the recommended standard hemostatic agent by the Committee on Tactical Combat Casualty Care (24). Many animal studies have shown the superiority of QuikClot in stopping hemorrhage compared to standard gauze. In a swine model of grade V liver injury, hemostasis was improved with 88% survival in the QuikClot group compared to standard gauze packing (23). Application is easy and fast, making it an ideal product in the field. However, due to the exothermic reaction of the product, there is a risk of burn injury. Patients often report heat and discomfort; one review examining 103 uses in the combat setting found that 3 patients suffered burns, with 1 severe enough to require skin grafting (25). Incorporating zeolites into mesh has decreased the incidence of this side-effect (20).
Chitin/chitosan-based products
Chitin, poly-N-acetyl glucosamine (pGlcNAc), is a component of fungal cell walls and the exoskeleton of arthropods with naturally occurring hemostatic properties (26). Chitin-based products work by firmly adhering to the wound, causing vasoconstriction and mobilizing erythrocytes, clotting factors, and platelets to the area of injury (27,28). Their mucoadhesive properties are ideal in that they can work independent of the physiological clotting mechanisms.
HemCon products contain chitosan, a deacetylated chitin, as their main ingredient. The product works like other chitin-based agents through its mucoadhesive properties. A variety of studies have shown its effectiveness and the product has been used with success in the recent conflicts in Afghanistan and Iraq (29,30). The most recent generation, ChitoGauze, is a highly flexible gauze dressing which has been found to be as effective as the other chitosan products and Combat Gauze (21,31).
The active ingredient in mRDH is poly-N-acetyl glucosamine, isolated from marine microalga (20). mRDH has demonstrated improved hemostasis in a variety of animal and human models. It was found to reduce mortality, blood loss and fluid requirements in a pig model of severe liver injury (32). In another study, mRDH bandages were used as packing for intra-abdominal trauma injuries. With the exception of one patient who died from another missed injury, all patients had cessation of bleeding with no re-bleeding after removal following re-exploration (33).
Celox is a chitosan derived from crab and shrimpshells (34). Its hemostatic action is due to interaction between the positively charged chitosan and negatively charged erythrocytes (35). Recent generations have incorporated the Celox into gauze, making it easier to apply in the field (34). Animal studies have shown significantly improved hemostasis in models of impaired coagulation including hypothermia and warfarin therapy (36). A number of case reports have demonstrated effective hemostasis clinically. One report of combat gunshot causalities in Afghanistan described successful hemostasis in all patients in the field using Celox products. After application of Celox, 18 of 21 wounds achieved hemostasis within 1 min. The remaining 3 wounds required additional Celox applications, but hemostasis was eventually achieved in 100% (37).
Biologically active agents
The coagulation cascade is a dynamic process involving the interplay between pro- and anti-thrombotic agents. With certain disease states, this complexity increases. For example, massive injury and shock from trauma leads to systemic anticoagulation and hyperfibrinolysis from a multi-factorial process involving tissue injury, decreased perfusion, hemodilution, acidemia and inflammation (38,39). Biologically inspired hemostatic agents must navigate this complex system to augment the natural hemostatic process at the site of injury without causing significant systemic side-effects.
Fibrin
Fibrin adhesives simulate the end products of the coagulation cascade and accelerate clot organization at the area of application. Commercially available fibrin adhesives vary in their physical properties and source components. The adhesive FloSeal is comprised of a combination of human thrombin solution and bovine fibrinogen gelatin granules that swell to generate a tamponade effect (40). Both human and animal studies have demonstrated the efficacy of these adhesives. In a study by Matthew et al. examining the use of fibrin adhesives in 689 cardiovascular and thoracic cases, the sealant was found to have a 94% success rate in controlling bleeding (41,42). In addition to hemostasis, fibrin sealants are clinically useful in colonic anastomoses and skin graft closures (43).
Thrombin
Topical thrombin works through the same pathway as native thrombin, converting fibrinogen to fibrin to form a stable clot, activating platelets and as a vasoconstrictor (44,45). It is available in bovine, human plasma, and recombinant human formulations. Topical thrombin is particularly useful in areas difficult to suture or cauterize, such as the spine, liver, or needle holes in polytetrafluoroethylene (PTFE) grafts. It also treats vascular pseudoaneurysms by causing local thrombosis when injected (46). Bovine thrombin has been utilized effectively for over 60 years in a wide variety of surgeries and organ systems, including peripheral artery bypass and arteriovenous grafting procedures (45,47). Reports of immune-mediated coagulopathy following exposure to bovine thrombin prompted the development of human and recombinant human thrombin products. However, up to 20% of patients who received bovine thrombin were found to have a higher incidence of antibodies against bovine thrombin (47,48). In some cases, the antibodies can cross-react with human coagulation factors which can cause severe bleeding or thrombosis (49). Despite the generation of antibodies, randomized-controlled phase III trials between bovine and human formulations have shown similar hemostatic efficacy and adverse outcomes (47,48).
Tranexamic acid (TA)
TA is a synthetic analogue of lysine and acts by blocking lysine-binding sites on plasminogen and preventing its activation. Intravenously injected TA functions as an antifibrinolytic agent. It is commonly used in cardiac surgery to reduce intraoperative bleeding and need for transfusion (50). Research has demonstrated that the administration of TA in trauma settings can reduce mortality by 17.4–23.9% (51). However, TA’s positive effects are dependent on administration time; TA given within the first three hours after traumatic incident considerably reduced the risk of hemorrhage fatality. Conversely, TA given after 3 h was demonstrated to be less effective and potentially harmful. This drug shows great promise in the treatment of traumatic hemorrhage and its addition to current resuscitation procedures is under consideration (52).
Recombinant factor VIIa (rFVIIa)
The intravenous use of rFVIIa as a mechanism for hemostatic control has been recently contested (42,53). In a study conducted by Boffard et al., the administration of rFVIIa in severe blunt trauma patients was found to significantly reduce the amount of massive transfusions required (54). Additionally, Rizoli et al. found that rFVIIa also resulted in a decrease of blood product utilization in coagulopathic patients (55). Conversely, it is unknown whether the administration of rFVIIa has any discernible effect on hemorrhagic trauma mortality rates. The use of rFVIIa in a pre-hospital setting has diminished due to its high costs, potentially harmful effects, and its difficulty to store (52).
Synthetic and cell-derived nanoparticles
Recent research has focused on engineering nanoparticles for a variety of clinical uses, including tissue adhesion, drug delivery and hemostasis (56). Cell-derived microparticles, such as thrombosomes, thromboerythrocytes, and synthocytes, have been evaluated as potential mechanisms for the treatment of bleeding. However, such particles were demonstrated to be ineffective at reducing bleeding and carry an added risk of adverse immunological response (57). Other nano-engineered agents have shown promise in both in vitro and animal models achieving hemostasis with reduced risk of undesired side-effects. Nanoparticle research is ongoing with both topical and intravenous agents (56).
Topical nanoparticles
Various engineered topical hemostatic agents have been trialed in vitro and with animal models. Ellis-Behnke et al. (58) described a model of self-assembling peptides. When applied to wound models of brain, spinal cord, femoral artery, liver and skin, complete hemostasis was achieved in less than 15 s, compared to a minimum 150 s among all organ models. Luo et al. (59) also described a model of self-assembling hemostatic nanofibers. The ionic peptides promoted rapid formation into nanofibers, forming a mesh structure resembling fish-netting. In a rabbit liver model, these molecules rapidly stopped bleeding much faster than no treatment, 17–20 s compared to 80–120 s. Gu et al. (60) developed a topical hemostat compromised of chitosan sheets. Nanofibers were created using electrospinning then neutralized to create an insoluble material. Its hemostatic action is due to the cation properties of chitosan and nonspecific cell membrane binding which promotes platelet aggregation and coagulation. In vitro, the nanofabricated chitosan fibers demonstrated 3.41-fold better platelet adhesion than a traditional chitosan sponge. Another topical hemostatic nanomolecule, engineered by Behrens et al. (61), employed cationic hydrogel particles based on N-(3-aminopropyl)methacrylamide with narrow size distribution and rapid swelling. In a rat liver and tail amputation model, a significant reduction in blood loss was observed, 300% and 500% respectively, compared to control gauze. The hydrogels also achieved hemostasis 1 min faster in the liver wound and 12 min faster in the tail wound (61).
Intravenous nanoparticles
A variety of engineered hemostatic intravenous agents have been described in the literature mimicking certain components of the platelet plug process (20,56). Synthetic liposome nanoparticles coated with various moieties have demonstrated improved hemostasis in lab models of bleeding (52,62-64). Attaching the dodecapeptide HHLGGAKQAGDV (H12) to the liposomal membrane demonstrated to restrain platelet aggregation in vivo, suggesting an interaction with activated platelets (52). Liposomes complexed with collagen binding peptides (CBP), vWF-binding peptides (VBP), and fibrinogen-mimetic peptides (FMP) also have been shown to promote platelet aggregation and reduce bleeding times in a mouse tail transection model by 50% (62).
Other groups have manufactured platelet-like molecules using synthetic molecular cores. Bertram et al. (65) developed synthetic platelets using a poly(lactic-co-glycolic acid)-poly-L-lysine (PLGA-PLL) block copolymer core with polyethylene glycol (PEG) arms terminated with Arg-Gly-Asp (RGD) functionalities (Figure 2). This model binds to activated platelets, enhancing aggregation. In a femoral artery injury model, bleeding time was halved following synthetic platelet administration (65). Nanoparticles using poly(lactic acid) (PLA) cores instead of PGLA were stable at temperatures reaching 50 °C and after stored for 7 days. This change allows for more translation to clinical applications (66). Anselmo et al. (9) manufactured nanoparticles to more closely mimic the physiological function of platelets. These platelet-like nanoparticles (PLN) exhibited similar biophysical and biochemical properties to natural platelets. The circumference of the PLN was comprised with poly(allylamine hydrochloride) and bovine serum albumin (PAH/BSA) bilayers, providing a flexible PAH/BSA shell to which CBP, VBP and FMP were attached. Their deformability mimics platelet malleability, allowing them to move from the initial binding to the site of vascular injury to spreading within fibrin matrices and finally to the contraction of a clot. The PLNs showed a bleeding time reduction of approximately 65% in a mouse-tail transection model. Further application to larger wounds and investigation into complement activation are needed (9).
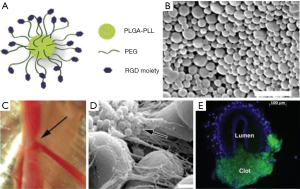
A recently developed molecule termed PolySTAT, crosslinks fibrin and markedly delays the time for clot fibrinolysis. PolySTAT increases affinity for fibrin through multiple binding domains. In a rat model, it reduced mortality with less blood loss and resuscitation fluid requirements (67). However, since PolySTAT depends on the presence of fibrin, it would be less effective in severe coagulopathy or deficiencies of the coagulation cascade. Translation of this technology to human trials may be possible in the future.
An element of the ideal intravenous hemostatic agent promotes clot formation at the site of injury without leading to potentially life-threatening thromboses elsewhere, such as the cerebral or coronary vasculatures. Using specific targets and elements unique to the active clotting cascade minimizes this risk. For example, the fibrin-specific binding of PolySTAT avoids systemic off-target clotting (67). PGLA-PLL synthetic polymers only bind to active platelets in the presence of ADP (65). The specificity of intravenous hemostats makes them attractive for further clinical development.
Conclusions
The ideal hemostatic agent is affordable, easily administered, stable, effective in a multitude of coagulopathies, and acts site-specific without immunogenic and pathologic thrombotic side-effects. A variety of agents are available for clinical use; a majority is topically applied. Deciding on which product to use depends on the bleed quality, location, and clinician preference. An active area of research surrounds nanoparticles, which have demonstrated site-specific clotting in vitro and in vivo. Translation of these studies to clinical trials is needed to demonstrate their efficacy and safety. The clinical use of targeted hemostatic agents has the potential to significantly reduce morbidity and mortality from hemorrhage, in particular for bleeds difficult to reach topically. This impact may be largest in traumatic hemorrhage, but could also have applications in chronically anticoagulated patients in whom reversal could cause unwanted thromboses.
Acknowledgements
Funding: R Oklu gratefully acknowledges funding from the National Institutes of Health (EB021148, CA172738, EB024403, HL137193) and the Mayo Clinic.
Footnote
Conflicts of Interest: The authors have no conflicts of interest to declare.
References
- Oklu R, Albadawi H, Jones JE, et al. Reduced hind limb ischemia-reperfusion injury in Toll-like receptor-4 mutant mice is associated with decreased neutrophil extracellular traps. J Vasc Surg 2013;58:1627-36. [Crossref] [PubMed]
- Sauaia A, Moore FA, Moore EE, et al. Epidemiology of trauma deaths: a reassessment. J Trauma 1995;38:185-93. [Crossref] [PubMed]
- Broderick J, Connolly S, Feldmann E, et al. Guidelines for the management of spontaneous intracerebral hemorrhage in adults: 2007 update: a guideline from the American Heart Association/American Stroke Association Stroke Council, High Blood Pressure Research Council, and the Quality of Care and Outcomes in Research Interdisciplinary Working Group. Stroke 2007;38:2001-23. [Crossref] [PubMed]
- Kim JW, Shin SS, Yoon W, et al. Diagnosis of acute gastrointestinal bleeding: comparison of the arterial, the portal, and the combined set using 64-section computed tomography. J Comput Assist Tomogr 2011;35:206-11. [Crossref] [PubMed]
- Semple JW, Italiano JE Jr, Freedman J. Platelets and the immune continuum. Nat Rev Immunol 2011;11:264-74. [Crossref] [PubMed]
- Cines DB, Pollak ES, Buck CA, et al. Endothelial cells in physiology and in the pathophysiology of vascular disorders. Blood 1998;91:3527-61. [PubMed]
- Palta S, Saroa R, Palta A. Overview of the coagulation system. Indian J Anaesth 2014;58:515-23. [Crossref] [PubMed]
- Broos K, Feys HB, De Meyer SF, et al. Platelets at work in primary hemostasis. Blood Reviews 2011;25:155-67. [Crossref] [PubMed]
- Anselmo AC, Modery-Pawlowski CL, Menegatti S, et al. Platelet-like nanoparticles: mimicking shape, flexibility, and surface biology of platelets to target vascular injuries. ACS Nano 2014;8:11243-53. [Crossref] [PubMed]
- Oklu R, Albadawi H, Watkins MT, et al. Detection of extracellular genomic DNA scaffold in human thrombus: implications for the use of deoxyribonuclease enzymes in thrombolysis. J Vasc Interv Radiol 2012;23:712-8. [Crossref] [PubMed]
- McMichael M. New Models of Hemostasis. Top Companion Anim Med. 2012;27:40-5. [Crossref] [PubMed]
- Morrissey JH, Tajkhorshid E, Sligar SG, et al. Tissue Factor/Factor VIIa Complex: Role of the Membrane Surface. Thromb Res 2012;129:S8-10. [Crossref] [PubMed]
- Perry DJ. Antithrombin and its inherited deficiencies. Blood Rev 1994;8:37-55. [Crossref] [PubMed]
- Ellery PE, Adams MJ. Tissue factor pathway inhibitor: then and now. Semin Thromb Hemost 2014;40:881-6. [Crossref] [PubMed]
- Esmon CT. The protein C pathway. Chest 2003;124:26s-32s. [Crossref] [PubMed]
- Hoylaerts M, Rijken DC, Lijnen HR, et al. Kinetics of the activation of plasminogen by human tissue plasminogen activator. Role of fibrin. J Biol Chem 1982;257:2912-9. [PubMed]
- Wicky S, Pinto EG, Oklu R. Catheter-directed thrombolysis of arterial thrombosis. Semin Thromb Hemost 2013;39:441-5. [Crossref] [PubMed]
- Ganguli S, Kalva S, Oklu R, et al. Efficacy of lower-extremity venous thrombolysis in the setting of congenital absence or atresia of the inferior vena cava. Cardiovasc Intervent Radiol 2012;35:1053-8. [Crossref] [PubMed]
- Oklu R, Wicky S. Catheter-directed thrombolysis of deep venous thrombosis. Semin Thromb Hemost 2013;39:446-51. [Crossref] [PubMed]
- Georgiou C, Neofytou K, Demetriades D. Local and systemic hemostatics as an adjunct to control bleeding in trauma. Am Surg 2013;79:180-7. [PubMed]
- Achneck HE, Sileshi B, Jamiolkowski RM, et al. A comprehensive review of topical hemostatic agents: efficacy and recommendations for use. Ann Surg 2010;251:217-28. [Crossref] [PubMed]
- Schwartz RB, Reynolds BZ, Shiver SA, et al. Comparison of two packable hemostatic Gauze dressings in a porcine hemorrhage model. Prehosp Emerg Care 2011;15:477-82. [Crossref] [PubMed]
- Pusateri AE, Delgado AV, Dick EJ Jr, et al. Application of a granular mineral-based hemostatic agent (QuikClot) to reduce blood loss after grade V liver injury in swine. J Trauma 2004;57:555-62; discussion 562. [Crossref] [PubMed]
- Kelly JF, Ritenour AE, McLaughlin DF, et al. Injury severity and causes of death from Operation Iraqi Freedom and Operation Enduring Freedom: 2003-2004 versus 2006. J Trauma 2008;64:S21-6; discussion S26-7.
- Rhee P, Brown C, Martin M, et al. QuikClot use in trauma for hemorrhage control: case series of 103 documented uses. J Trauma 2008;64:1093-9. [Crossref] [PubMed]
- Kulling D, Vournakis JN, Woo S, et al. Endoscopic injection of bleeding esophageal varices with a poly-N-acetyl glucosamine gel formulation in the canine portal hypertension model. Gastrointest Endosc 1999;49:764-71. [Crossref] [PubMed]
- Kheirabadi BS, Edens JW, Terrazas IB, et al. Comparison of new hemostatic granules/powders with currently deployed hemostatic products in a lethal model of extremity arterial hemorrhage in swine. J Trauma 2009;66:316-26; discussion 327-8. [Crossref] [PubMed]
- Ikeda Y, Young LH, Vournakis JN, et al. Vascular effects of poly-N-acetylglucosamine in isolated rat aortic rings. J Surg Res 2002;102:215-20. [Crossref] [PubMed]
- Bennett BL, Littlejohn L. Review of new topical hemostatic dressings for combat casualty care. Mil Med 2014;179:497-514. [Crossref] [PubMed]
- Wedmore I, McManus JG, Pusateri AE, et al. A special report on the chitosan-based hemostatic dressing: experience in current combat operations. J Trauma 2006;60:655-8. [Crossref] [PubMed]
- Devlin JJ, Kircher S, Kozen BG, et al. Comparison of ChitoFlex(R), CELOX, and QuikClot(R) in control of hemorrhage. J Emerg Med 2011;41:237-45. [PubMed]
- Jewelewicz DD, Cohn SM, Crookes BA, et al. Modified rapid deployment hemostat bandage reduces blood loss and mortality in coagulopathic pigs with severe liver injury. J Trauma 2003;55:275-80; discussion 280-1. [Crossref] [PubMed]
- King DR, Cohn SM, Proctor KG, et al. Modified rapid deployment hemostat bandage terminates bleeding in coagulopathic patients with severe visceral injuries. J Trauma 2004;57:756-9. [Crossref] [PubMed]
- Millner RW, Lockhart AS, Bird H, et al. A new hemostatic agent: initial life-saving experience with Celox (chitosan) in cardiothoracic surgery. Ann Thorac Surg 2009;87:e13-4. [Crossref] [PubMed]
- Rao SB, Sharma CP. Use of chitosan as a biomaterial: studies on its safety and hemostatic potential. J Biomed Mater Res 1997;34:21-8. [Crossref] [PubMed]
- Koksal O, Ozdemir F, Cam Etoz B, et al. Hemostatic effect of a chitosan linear polymer (Celox(R)) in a severe femoral artery bleeding rat model under hypothermia or warfarin therapy. Ulus Travma Acil Cerrahi Derg 2011;17:199-204. [Crossref] [PubMed]
- Pozza M, Millner RW. Celox (chitosan) for haemostasis in massive traumatic bleeding: experience in Afghanistan. Eur J Emerg Med 2011;18:31-3. [Crossref] [PubMed]
- Hess JR, Brohi K, Dutton RP, et al. The Coagulopathy of Trauma: A Review of Mechanisms. J Trauma 2008;65:748-54. [Crossref] [PubMed]
- Brohi K. Make the bleeding stop. Sci Transl Med 2015;7:277fs10. [Crossref] [PubMed]
- Oz MC, Rondinone JF, Shargill NS. FloSeal Matrix: new generation topical hemostatic sealant. J Card Surg 2003;18:486-93. [Crossref] [PubMed]
- Matthew TL, Spotnitz WD, Kron IL, et al. Four years' experience with fibrin sealant in thoracic and cardiovascular surgery. Ann Thorac Surg 1990;50:40-3; discussion 43-4. [Crossref] [PubMed]
- Recinos G, Inaba K, Dubose J, et al. Local and systemic hemostatics in trauma: a review. Ulus Travma Acil Cerrahi Derg 2008;14:175-81. [PubMed]
- Spotnitz WD. Fibrin Sealant: Past, Present, and Future: A Brief Review. World J Surg 2010;34:632-4. [Crossref] [PubMed]
- Lomax C, Traub O. Topical thrombins: benefits and risks. Pharmacotherapy 2009;29:8S-12S. [Crossref] [PubMed]
- Lawson JH. The clinical use and immunologic impact of thrombin in surgery. Semin Thromb Hemost 2006;32 Suppl 1:98-110. [Crossref] [PubMed]
- Stone PA, Campbell JE, AbuRahma AF. Femoral pseudoaneurysms after percutaneous access. J Vasc Surg 2014;60:1359-66. [Crossref] [PubMed]
- Chapman WC, Singla N, Genyk Y, et al. A Phase 3, Randomized, Double-Blind Comparative Study of the Efficacy and Safety of Topical Recombinant Human Thrombin and Bovine Thrombin in Surgical Hemostasis. J Am Coll Surg 2007;205:256-65. [Crossref] [PubMed]
- Doria C, Fischer CP, Wood CG, et al. Phase 3, randomized, double-blind study of plasma-derived human thrombin versus bovine thrombin in achieving hemostasis in patients undergoing surgery. Curr Med Res Opin 2008;24:785-94. [Crossref] [PubMed]
- Gabay M, Boucher BA. An essential primer for understanding the role of topical hemostats, surgical sealants, and adhesives for maintaining hemostasis. Pharmacotherapy 2013;33:935-55. [Crossref] [PubMed]
- Myles PS, Smith JA, Forbes A, et al. Tranexamic Acid in Patients Undergoing Coronary-Artery Surgery. N Engl J Med 2017;376:136-48. [Crossref] [PubMed]
- Hunt BJ. The current place of tranexamic acid in the management of bleeding. Anaesthesia 2015;70 Suppl 1:50-3, e18.
- Lashof-Sullivan M, Shoffstall A, Lavik E. Intravenous hemostats: challenges in translation to patients. Nanoscale 2013;5:10719-28. [Crossref] [PubMed]
- Kenet G, Walden R, Eldad A, et al. Treatment of traumatic bleeding with recombinant factor VIIa. Lancet 1999;354:1879. [Crossref] [PubMed]
- Boffard KD, Riou B, Warren B, et al. Recombinant factor VIIa as adjunctive therapy for bleeding control in severely injured trauma patients: two parallel randomized, placebo-controlled, double-blind clinical trials. J Trauma 2005;59:8-15; discussion 15-8. [Crossref] [PubMed]
- Rizoli SB, Boffard KD, Riou B, et al. Recombinant activated factor VII as an adjunctive therapy for bleeding control in severe trauma patients with coagulopathy: subgroup analysis from two randomized trials. Crit Care 2006;10:R178. [Crossref] [PubMed]
- Annabi N, Tamayol A, Shin SR, et al. Surgical Materials: Current Challenges and Nano-enabled Solutions. Nano Today 2014;9:574-89. [Crossref] [PubMed]
- Shoffstall AJ, Atkins KT, Groynom RE, et al. Intravenous hemostatic nanoparticles increase survival following blunt trauma injury. Biomacromolecules 2012;13:3850-7. [Crossref] [PubMed]
- Ellis-Behnke RG, Liang YX, Tay DK, et al. Nano hemostat solution: immediate hemostasis at the nanoscale. Nanomedicine 2006;2:207-15. [Crossref] [PubMed]
- Luo Z, Wang S, Zhang S. Fabrication of self-assembling d-form peptide nanofiber scaffold d-EAK16 for rapid hemostasis. Biomaterials 2011;32:2013-20. [Crossref] [PubMed]
- Gu BK, Park SJ, Kim MS, et al. Fabrication of sonicated chitosan nanofiber mat with enlarged porosity for use as hemostatic materials. Carbohydr Polym 2013;97:65-73. [Crossref] [PubMed]
- Behrens AM, Sikorski MJ, Li T, et al. Blood-aggregating hydrogel particles for use as a hemostatic agent. Acta Biomaterialia 2014;10:701-8. [Crossref] [PubMed]
- Modery-Pawlowski CL, Tian LL, Ravikumar M, et al. In vitro and in vivo hemostatic capabilities of a functionally integrated platelet-mimetic liposomal nanoconstruct. Biomaterials 2013;34:3031-41. [Crossref] [PubMed]
- Ravikumar M, Modery CL, Wong TL, et al. Mimicking adhesive functionalities of blood platelets using ligand-decorated liposomes. Bioconjug Chem 2012;23:1266-75. [Crossref] [PubMed]
- Lashof-Sullivan MM, Shoffstall E, Atkins KT, et al. Intravenously administered nanoparticles increase survival following blast trauma. Proc Natl Acad Sci U S A 2014;111:10293-8. [Crossref] [PubMed]
- Bertram JP, Williams CA, Robinson R, et al. Intravenous hemostat: nanotechnology to halt bleeding. Sci Transl Med 2009;1:11ra22. [Crossref] [PubMed]
- Lashof-Sullivan M, Holland M, Groynom R, et al. Hemostatic Nanoparticles Improve Survival Following Blunt Trauma Even after 1 Week Incubation at 50 degrees C. ACS Biomater Sci Eng 2016;2:385-92. [Crossref] [PubMed]
- Chan LW, Wang X, Wei H, et al. A synthetic fibrin cross-linking polymer for modulating clot properties and inducing hemostasis. Sci Transl Med 2015;7:277ra29. [Crossref] [PubMed]