Myocardial T1 mapping: modalities and clinical applications
Introduction
There is increasing evidence that the underlying, pathological basis for a variety of non-ischemic cardiomyopathies is myocardial fibrosis. This pathological process of extracellular matrix (ECM) remodeling results in multiple functional consequences including impaired relaxation (1), precipitation of arrhythmias (2) and impaired contractile reserve (3). Traditionally, myocardial fibrosis has been recognized in conditions such as hypertensive heart disease, hypertrophic cardiomyopathy (HCM) and idiopathic dilated cardiomyopathy (DCM). However, there is increasing identification of myocardial fibrosis as the common causative factor of myocardial dysfunction in many other, particularly metabolic, processes such as obesity, diabetes and aging. Early identification of this underlying, often diffuse, interstitial myocardial fibrosis is important for diagnosis and essential for optimization of reversibility with intervention. Multiple non-invasive diagnostic strategies have been trialed as an alternative to endomyocardial biopsy for fibrosis assessment including: echocardiographic backscatter (4), nuclear imaging (5), peripheral collagen biomarkers (6) and molecular labeling techniques (7). Their clinical application has remained limited. The rapid development of cardiac magnetic resonance (CMR) imaging heralds a new era of promising techniques for identification of ECM accumulation within the heart and surrounding structures. Delayed gadolinium enhancement (DE-CMR) imaging has become the new gold standard for identification of focal fibrosis. T1 mapping has the potential to provide a similar quantitative assessment of diffuse myocardial fibrosis.
Evolution of fibrosis quantification by CMR
The ability to achieve high resolution, 3-dimensional imaging without the use of ionizing radiation has ushered in an age of safer and superior tissue characterization. Magnetic resonance imaging exploits the magnetic properties of hydrogen nuclei protons within a determined magnetic field. Longitudinal relaxation time (T1) and transverse relaxation time (T2) are central properties. T1 and T2 are determined by the molecular make-up of tissue, which is primarily determined by the proportion of water content. The T1 value is specifically defined as the time when longitudinal proton magnetization recovers approximately 63% of its equilibrium value (8).
DE-CMR imaging has been widely used to detect and assess myocardial scar and perfusion. It is the non-invasive gold standard for quantification of focal myocardial fibrosis as small as 0.16 g (9) and is based on the premise that there is a distribution difference of contrast between normal and fibrotic myocardium. Areas of fibrosis demonstrate greater gadolinium accumulation which is represented as a region of high intensity signal with a shorter T1 time than adjacent normal tissue (10). This is clinically useful for assessment of infarction and other regional processes such as the mid-myocardial fibrosis of HCM (11). Conventionally this method is performed using inversion recovery gradient-echo sequences 10-15 minutes after gadolinium infusion. Retention of contrast within the extracellular space results in shortening of the inversion time (T1) and hyperenhancement relative to normal myocardium. Ischemic scar usually results in delayed enhancement (DE) in a subendocardial or transmural distribution consistent with the perfusion territories of epicardial coronary arteries, while non-ischemic fibrosis tends to be irregular and intramural or subepicardial in distribution (12).
The main limiting factor with using DE-CMR in non-ischemic cardiomyopathies is that the fibrotic process is often diffuse, thereby lacking the presence of normal non-fibrotic myocardium as a frame of reference. This can result in underestimation of the true expanded ECM burden due to interstitial fibrosis. To ameliorate this issue, contrast enhanced T1 mapping has been developed to quantify diffuse, non-ischemic myocardial fibrosis, as it does not rely on contrasting signal intensity. It is an evolving technique, which has also needed to overcome the inherent technical issues associated with physiological cardiac and respiratory motion.
T1 mapping techniques
Multiple CMR T1 based techniques have been employed for quantitation of diffuse fibrosis. Currently all have inherent advantages and limitations. Messroghli et al. have been at the forefront of T1 mapping sequence development (13). Their modified Look Locker inversion recovery (MOLLI) technique was designed to overcome the limitations of motion and prolonged acquisition time. Specifics included an ability to acquire data within one breath-hold at a designated time within the cardiac cycle and the capability of merging images from multiple Look Locker experiments at different consecutive inversion times into one data set. MOLLI sequencing employs a balanced steady-state free precession (SSFP) readout to achieve a higher signal to noise ratio with a narrow image acquisition period of less than 200 msec in end-diastole to minimize motion artifact (13). Reproducibility of this technique is high (14). Even faster acquisition times have been achieved by Piechnik et al. who developed a shortened MOLLI sequence (ShMOLLI) which requires a short breath-hold of only nine heart beats duration (15). Both sequences are similar but ShMOLLI does not require full recovery of magnetization on sequential inversion pulses and has less heart rate (HR) dependency, which may improve accuracy (16). Other prototype vendor specific sequences are in development including a modified Look-Locker FIESTA technique using saturation-recovery imaging (17), saturation-recovery single shot acquisition (SASHA) (18), and saturation pulse prepared heart-rate-independent inversion recovery, which consists of a combination of saturation and inversion pulses (19).
T1 mapping methodology
There are variations on the exact methodology employed by the various rapid T1 mapping sequences to determine T1 time. Current protocols are increasingly using both pre- and post-contrast imaging (Figure 1). Typically a series of short-axis images are acquired pre- and approximately 12 minutes after gadolinium-based contrast with between 6 and 12 consecutively longer inversion times depending on the protocol. Image acquisition is best performed with vectorcardiogram (VCG) gating and the use of inspiratory breath holds. Offline, standard basal, mid-ventricular and/or apical slices are then selected and can be further divided into conventional myocardial segments (or discrete regions of interest) and blood-pool for analysis if desired. A curve fitting technique is employed to reconstruct the consecutively acquired images into one data set from which a T1 map of voxels is generated. The signal intensity of each mapped voxel directly represents the T1 relaxation time of the corresponding myocardial tissue. An exponential recovery curve of signal intensities at the different inversion times is then created for each designated region of interest (slice or segment) to determine the myocardial T1 relaxation time. These can be averaged to extrapolate T1 as an index of diffuse fibrosis, where shorter T1 time and higher signal intensity correspond to increased gadolinium contrast accumulation within the ECM (Figure 2).
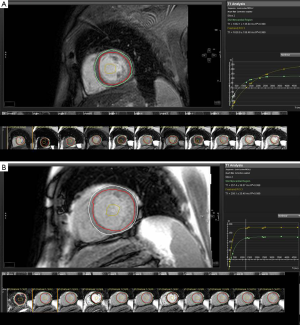
Standardization of myocardial T1 values to non-myocardial tissue (blood) assists in minimizing the dynamic features of gadolinium contrast. The ratio of myocardial to blood post-contrast T1 values is expressed as the partition coefficient. By preceding post-contrast T1 mapping with pre-contrast T1 mapping, myocardial extra-cellular volume (ECV) can similarly be derived. This pseudo-equilibrium technique employs the reciprocals of myocardial and blood T1 values pre- and post-contrast and then adjusts for hematocrit to correct for the blood contrast volume of distribution (20,21). This ECV is expressed as a percentage of the total myocardial extracellular space. Gadolinium equilibrium between blood and myocardium must be achieved prior to image acquisition to enable accurate estimation of ECV. Typically this occurs from 8.5 minutes post contrast injection, after which ECV differences between time-points are minimal (22). A larger ECV represents increased contrast accumulation in an expanded ECM.
T1 mapping advantages and limitations
Pre-contrast/native T1 mapping
Pre-contrast techniques measure the intrinsic or native myocardial T1. They have an inherent advantage for subjects with significant renal impairment who may be at increased risk of nephrogenic systemic sclerosis. However, non-contrast T1 mapping is limited by an inability to clearly distinguish interstitial from myocyte signal intensity. This may be of clinical consequence in early disease processes when there is minimal difference in T1 values between healthy and disease states. Accuracy is also reduced at longer T1 values. As noted above, HR is a documented physiologic covariate of T1 values due to imaging dependency on VCG triggering (14). This is especially problematic when imaging at high magnetic field strengths (3T). Currently, standard Look-Locker and rapid T1 mapping sequences do not have in-built features for HR correction. However, some of the newer techniques such as ShMOLLI have faster acquisition times and are inherently less HR dependent. Standardization of the acquisition phase in the cardiac cycle within and between studies remains an important consideration as acquisition timing can impact on T1 value and ECV due to fluctuation of the myocardial blood volume (23). A reduction in T1 time of 43-70% has been observed from diastole to systole (24). Clinical feasibility of T1 mapping markedly increased with the advent of non-contrast MOLLI imaging due to increased reproducibility, higher spatial resolution and reduced motion artifact. However, this technique remains prone to frequency-dependent errors in T1-measurement (25), which has limited its practical utility.
Post-contrast
Post-contrast T1 mapping sequences are relatively intuitive for those familiar with CMR and can be readily incorporated into standard DE-CMR protocols without significant prolongation of study duration. In addition to HR and acquisition related confounders, there are several established physiological covariates related to contrast administration, which must be considered. These include: body fat percentage, renal function, delay time in measurement after contrast administration and gadolinium characteristics (such as dose, concentration and water exchange rate). Contrast dispersion may be influenced by higher percentage body fat composition in some individuals, leading to increased contrast dose relative to extra-cellular water content. Contrast administration is relatively contraindicated in subjects with an estimated glomerular filtration rate of <30 mL/min/1.73 m2. Ideally, all confounding factors should be screened as predictors of post-contrast T1 values using linear regression analysis to enable appropriate data correction prior to analysis (26).
Although acquisition of pre- and post-contrast T1 mapping images can be performed in a timely fashion using the current rapid protocols, offline post-processing still remains laborious. Due to the time consuming nature of analysis of T1 values in multiple segments, some studies have also limited assessment to single mid-ventricular regions of interest and assumed these to be representative of the whole myocardium. Segmental analysis can also be problematic due to ventricular motion artifacts which occur most frequently in the infero-postero-lateral region (17). These regions may need to be deemed non-evaluable and excluded from analysis.
Partition coefficient and ECV mapping
Estimation of partition coefficient and ECV fraction from T1 mapping reduce the effect of confounding variables as they are calculated from the ratio of change in myocardial T1 relative to blood-pool T1 pre and post-contrast (27). The advantage of this type of methodology is that it minimizes systematic errors in technique, enables better comparison of scans at different time points and results in less variability at different field strengths and across different vendor platforms. This could be of significant clinic benefit for monitoring of interval change within individuals, assessing treatment effect and allowing comparison of disease patterns within the general community. The main confounding factor affecting the partition coefficient is hematocrit. However, it can be easily corrected using blood volume of distribution (1-hematocrit) to give the ECV. Although the ECV is a more reliable measure of interstitial space, it remains based on the assumption of contrast equilibrium between blood and myocardium. Theoretically this may be problematic in certain disease states, although practically this does not appear to be an issue provided there is an adequate time period allowed for adequate contrast dispersion (22). Clinically, there is a wide spread of ECV values with overlap of values between normal and diseased myocardium (23). This makes it problematic for diagnostic purposes and more suited to measurement of interval change within individuals or assessment of more end-stage disease. Controversy persists regarding the influence of magnetic field strength (1.5 vs. 3 T) on myocardial ECV (22,23).
Alternative volume of distribution T1 mapping techniques
In an attempt to negate the inherent limitations of T1 mapping described above, alternative CMR techniques using volume of distribution methodology have been developed to estimate myocardial ECM burden. Equilibrium contrast cardiovascular magnetic resonance imaging (EQ-CMR) relies on a primed continuous infusion of contrast to achieve blood: myocardial equilibrium and thereby negate the effects of kinetic confounders (28). The three pronged method involves a contrast bolus followed by infusion, a blood sample to measure blood volume of distribution (1-hematocrit) and T1 measures pre- and post-equilibrium (with HR correction) to calculate the myocardial volume of distribution as a measure of diffuse myocardial fibrosis (28). Results are promising, however, widespread adoption of this technique outside research protocols has been limited due to its time and labor consuming methodology. Attempts have been made to simplify this technique with bolus contrast administration, however, this results in a disparity with infusion ECV results when ECV levels are >0.4 (29). Volume of distribution has also been estimated via mathematical modeling. Jerosh-Herold et al. describe a technique whereby they perform multiple T1 measures during contrast washout and use complex mathematical modeling to minimize the effects of kinetic confounders and establish the ECV (27). The practical aspects of this technique are much simpler than EQ-CMR, meaning that it could be more easily integrated into a busy CMR lab. However, due to the multiple (and often exponential) factors, which determine contrast washout, mathematical modeling is complex and potentially liable to multiple predictable and unpredictable limitations. This may reduce its accuracy in ECV quantification as small calculation errors may be multiplied by several factors of magnitude in complex equations.
Technique validation
ECM is synthesized and degraded in a dynamic process of continual tissue growth and repair. This structural scaffolding is constituted in part from fibrillar collagen [predominantly types I (30) and III (31)], which provides both strength and elasticity. However, certain factors predispose to detrimental myocardial ECM remodeling, including: activation of inflammatory cytokines (32), potentiation of neurohormonal cascades (33), and upregulation of the renin-angiotensin-aldosterone system (34). Initial clinical T1 mapping studies examined advanced disease states where fibrosis had a known underling pathological role. Iles et al. studied a symptomatic heterogeneous heart failure population, which included transplant recipients. They used post-contrast MOLLI to demonstrate an inverse correlation of T1 values with percentage fibrosis on myocardial biopsy of transplanted hearts, as well as a reduction in T1 time with worsening diastolic function (35). These results were corroborated by Sibley et al. who used a post-contrast Look-Locker technique to document an inverse correlation between T1 time and histological fibrosis on biopsy in patients with a broad range of cardiomyopathies, regardless of the degree of DE-CMR (36). However, in both these studies, endomyocardial biopsy samples were taken from the right ventricle, whilst T1 values were taken from left ventricular maps on the assumption that the pathological process was one of diffuse fibrosis.
A small number of other studies employing post-contrast Look-Locker, MOLLI and other T1 techniques have demonstrated a correlation between T1 values and percentage myocardial fibrosis in a variety of pathologies. Non-contrast T1 mapping techniques have been less comprehensively compared against histological samples. ShMOLLI is the best validated and has demonstrated a relationship between T1 values and ECV fraction in severe aortic stenosis (37). Histological validation of the volume of distribution method EQ-CMR has been performed in small numbers of patients with aortic stenosis (28). Histological validation of T1 mapping techniques in early disease states is lacking due to the obvious ethical issues associated with performing invasive biopsies on relatively well subjects. Correlation of T1 values with collagen volume fraction in small animal models (20) has provided some indirect methodological validation (Table 1). While validation with histopathology is of considerable importance, the use of histopathology as the gold standard may also have its limitations. Significant desiccation of tissue samples frequently occurs during the fixation process, which can alter ECV, which may explain way many groups have not been able to demonstrate r values >0.7. Therefore, validation with hard clinical endpoints and the demonstration of T1mapping to predict response to various treatments will be necessary to launch T1mapping into mainstream clinical use.
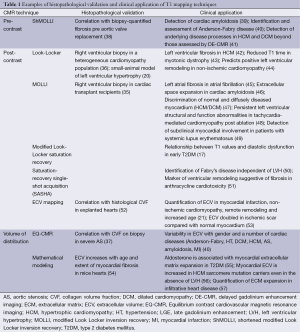
Full table
Clinical applications
T1 values have been correlated with echocardiographic functional and structural parameters, as well as pathological processes, which are known to be associated with increased myocardial fibrosis. T1 mapping has also been employed in subclinical myocardial disease to determine if underlying functional disturbance detected on echocardiographic parameters correlates with reduced T1 values in asymptomatic subjects in whom myocardial biopsy cannot be ethically justified. Markers of both systolic (58) and diastolic dysfunction (17) have been found to correlate with reduced T1 values in early non-ischemic diabetic heart disease suggesting a possible pathological role of fibrosis even in early disease states. This is reinforced by the study by Rao et al., which found aldosterone excess to be associated with ECM expansion in T2DM (55) (Table 1). Thus, further studies are needed to determine if treating patients with early disease states can prevent progression to more advanced disease. In addition, T1mapping has the potential to risk stratify patients with advanced cardiac disease and identify patients who may not respond to medical therapy and should be treated with more aggressive interventions such as device therapies.
Broberg et al. demonstrated a relationship between increased ECV and worsening of volumetric parameters in subjects with adult congenital heart disease compared to controls (59). Myocardial ECV has also been shown to correlate with short-term mortality (60) and histological collagen burden with low variability between scans (61). It has recently been found to be proportionally increased with advancement of age (21) and female gender (62). It must always be remembered that a reduction in T1 values is not specific for fibrosis and can be noted in alternative infiltrative pathologies, which cause increased myocardial signal intensity such as cardiac amyloid (63). However, these processes often demonstrate characteristic patterns of distribution, which can already be appreciated and differentiated using DE-CMR. The impact of myofibril disarray on T1 mapping in HCM remains controversial but may result in overestimation of ECV (64).
Although T1 mapping is typically employed for assessment of diffuse, non-ischemic fibrosis, it has been conversely used for characterization of myocardial infarction including Tako-tsubo cardiomyopathy (65). Acutely, prolongation of T1 and T2 values occurs in the setting of increased free water molecules (66). Increased pre-contrast T1 values not only reflect greater edema suggestive of more severe cellular injury (65), but also suggest a reduced likelihood of improvement in myocardial function long-term (67). This same premise shows promise for detection of non-ischemic acute edema in conditions such as myocarditis (65). Chronically, reduced T1 values have been demonstrated in regional infarcted tissue compared with normal myocardium (16).
Future directions
Assessment of ECM expansion in the setting of valve disease with T1 mapping has remained restricted to small cohorts with aortic valve disease (37,38,40,68). However, the potential clinical applications are immense, especially given the increasing body of evidence demonstrating a direct relationship between fibrosis burden and adverse outcomes (69). Quantification of diffuse fibrosis may assist with timing of surgery and predicting response to and recovery from surgical interventions (i.e., valve replacement/repair) in a similar way to the use of contractile reserve with stress echocardiography. Current research studies exploring T1 mapping in these areas will hopefully further add to the potential clinical applications of this evolving technique.
As the technique of T1 mapping matures, serial monitoring of other non-valvular disease states such as HCM will hopefully also clarify the natural history of fibrosis progression. This may assist in the timing of targeted interventions. Patterns of diffuse fibrosis may also become more evident in a similar way to the characteristic changes observed on DE-CMR. Although, achieving similar diagnostic sensitivities to DE-CMR will be difficult. T1 mapping should also be well suited to identification of more focal regions of diffuse fibrosis. In real world clinical practice, it is likely that many subjects will present with concurrent manifestations of both diffuse and focal fibrosis. A common anticipated example would be ischemic cardiomyopathy in the setting of diabetes or obesity. This overlap may prove challenging for T1 quantification, as the degree of regional contrast accumulation will significantly impact upon the global T1 value (70). Accounting for these regions of focal enhancement by excluding them from T1 maps may assist, but would be technically difficult using current manual techniques. Future development of graded maps of signal intensity may enable automatic exclusion of regions with T1 values outside nominated ranges from global T1 calculations.
Accuracy of T1 values remains to be conclusively established. Currently, this is limiting its widespread use as a clinical application for diagnosis and guiding treatment. Histopathological validation remains limited and needs to be significantly improved across all T1 modalities. This is crucial for determination of accuracy as well as establishing the relationship of T1 values from different sequences, vendors and field strengths. Thus far, this type of multimodality testing has been largely limited to phantom studies (71). Overall, test-retest reproducibility appears to be good (54), especially with the newer single breath-hold ShMOLLI technique (37,72). However, larger, multicenter trials will be important to establish inter-observer variability between institutions and different T1 mapping techniques. Similarly, reliability of documented normal valves for both T1 and ECV remains suboptimal due to limited studies with small subject numbers (54). Once definitive normal valves are established, diagnostic valves in disease states will be more reliable and clinically meaningful. The recently described method of predicting T1 mapping and ECV measurement error may enable more confident identification of early disease as well as facilitate sequence optimization and allow more direct comparison of different T1 mapping protocols (25). Lastly, even the most rapid T1 sequences are limited by lengthy post-processing. Development of reliable tissue tracking and automated border detection software for delineation of regions of interest would significantly improve the functionality and clinical applicability of the technique.
Conclusions
T1 mapping has the potential to be a useful technique for quantification of diffuse myocardial fibrosis in a variety of clinical settings. Currently, limited accessibility, accuracy, clear reference values for normal and abnormal myocardium and uniformity of technique amongst vendor specific sequences impede its practical applicability. Until currently, multiple research groups are actively addressing these issues, as the number of publications with T1mapping has exponentially increased over the past few years, and the field is rapidly expanding. Multiple centers continue to apply various T1 mapping techniques to characterize various disease states which will undoubtedly provide the basis for more widespread clinical applicability. While our ability to interpret and compare meaningful data regarding true fibrosis burden from T1 values is currently limited, the advent of rapid T1mapping for mainstream clinical use is likely to arrive in the not to distant future.
Acknowledgements
Disclosure: The authors declare no conflict of interest.
References
- Sugihara N, Genda A, Shimizu M, et al. Diastolic dysfunction and its relation to myocardial fibrosis in essential hypertension. J Cardiol 1988;18:353-61. [PubMed]
- Ling LH, Kistler PM, Ellims AH, et al. Diffuse ventricular fibrosis in atrial fibrillation: noninvasive evaluation and relationships with aging and systolic dysfunction. J Am Coll Cardiol 2012;60:2402-8. [PubMed]
- McLenachan JM, Dargie HJ. Ventricular arrhythmias in hypertensive left ventricular hypertrophy. Relationship to coronary artery disease, left ventricular dysfunction, and myocardial fibrosis. Am J Hypertens 1990;3:735-40. [PubMed]
- Picano E, Pelosi G, Marzilli M, et al. In vivo quantitative ultrasonic evaluation of myocardial fibrosis in humans. Circulation 1990;81:58-64. [PubMed]
- Knaapen P, Boellaard R, Götte MJ, et al. Perfusable tissue index as a potential marker of fibrosis in patients with idiopathic dilated cardiomyopathy. J Nucl Med 2004;45:1299-304. [PubMed]
- Díez J, Panizo A, Gil MJ, et al. Serum markers of collagen type I metabolism in spontaneously hypertensive rats: relation to myocardial fibrosis. Circulation 1996;93:1026-32. [PubMed]
- van den Borne SW, Diez J, Blankesteijn WM, et al. Myocardial remodeling after infarction: the role of myofibroblasts. Nat Rev Cardiol 2010;7:30-7. [PubMed]
- Hwang SH, Choi BW. Advanced Cardiac MR Imaging for Myocardial Characterization and Quantification: T1 Mapping. Korean Circ J 2013;43:1-6. [PubMed]
- Wu E, Judd RM, Vargas JD, et al. Visualisation of presence, location, and transmural extent of healed Q-wave and non-Q-wave myocardial infarction. Lancet 2001;357:21-8. [PubMed]
- Dulce MC, Duerinckx AJ, Hartiala J, et al. MR imaging of the myocardium using nonionic contrast medium: signal-intensity changes in patients with subacute myocardial infarction. AJR Am J Roentgenol 1993;160:963-70. [PubMed]
- Kwon DH, Desai MY. Cardiac magnetic resonance in hypertrophic cardiomyopathy: current state of the art. Expert Rev Cardiovasc Ther 2010;8:103-11. [PubMed]
- Gottlieb I, Macedo R, Bluemke DA, et al. Magnetic resonance imaging in the evaluation of non-ischemic cardiomyopathies: current applications and future perspectives. Heart Fail Rev 2006;11:313-23. [PubMed]
- Messroghli DR, Radjenovic A, Kozerke S, et al. Modified Look-Locker inversion recovery (MOLLI) for high-resolution T1 mapping of the heart. Magn Reson Med 2004;52:141-6. [PubMed]
- Messroghli DR, Plein S, Higgins DM, et al. Human myocardium: single-breath-hold MR T1 mapping with high spatial resolution--reproducibility study. Radiology 2006;238:1004-12. [PubMed]
- Piechnik SK, Ferreira VM, Dall’Armellina E, et al. Shortened modified look-locker inversion recovery (shmolli) for clinical myocardial t1-mapping at 1.5 and 3 T within a 9 heartbeat breathhold. J Cardiovasc Magn Reson 2010;12:69. [PubMed]
- Messroghli DR, Greiser A, Frohlich M, et al. Optimization and validation of a fully-integrated pulse sequence for modified look-locker inversion-recovery (molli) T1 mapping of the heart. J Magn Reson Imaging 2007;26:1081-6. [PubMed]
- Jellis C, Wright J, Kennedy D, et al. Association of imaging markers of myocardial fibrosis with metabolic and functional disturbances in early diabetic cardiomyopathy. Circ Cardiovasc Imaging 2011;4:693-702. [PubMed]
- Chow K, Flewitt JA, Green JD, et al. Saturation recovery single-shot acquisition (SASHA) for myocardial T1 mapping. Magn Reson Med 2013. [Epub ahead of print]. [PubMed]
- Weingärtner S, Akçakaya M, Basha T, et al. Combined saturation/inversion recovery sequences for improved evaluation of scar and diffuse fibrosis in patients with arrhythmia or heart rate variability. Magn Reson Med 2013. [Epub ahead of print]. [PubMed]
- Messroghli DR, Nordmeyer S, Dietrich T, et al. Assessment of diffuse myocardial fibrosis in rats using small-animal look-locker inversion recovery T1 mapping. Circ Cardiovasc Imaging 2011;4:636-40. [PubMed]
- Ugander M, Oki AJ, Hsu LY, et al. Extracellular volume imaging by magnetic resonance imaging provides insights into overt and sub-clinical myocardial pathology. Eur Heart J 2012;33:1268-78. [PubMed]
- Lee JJ, Liu S, Nacif MS, et al. Myocardial T1 and extracellular volume fraction mapping at 3 tesla. J Cardiovasc Magn Reson 2011;13:75. [PubMed]
- Kawel N, Nacif M, Zavodni A, et al. T1 mapping of the myocardium: intra-individual assessment of post-contrast t1 time evolution and extracellular volume fraction at 3T for gd-dtpa and gd-bopta. J Cardiovasc Magn Reson 2012;14:26. [PubMed]
- Wansapura J, Gottliebson W, Crotty E, et al. Cyclic variation of T1 in the myocardium at 3T. Magn Reson Imaging 2006;24:889-93. [PubMed]
- Kellman P, Arai AE, Xue H. T1 and extracellular volume mapping in the heart: estimation of error maps and the influence of noise on precision. J Cardiovasc Magn Reson 2013;15:56. [PubMed]
- Gai N, Turkbey EB, Nazarian S, et al. T1 mapping of the gadolinium-enhanced myocardium: adjustment for factors affecting interpatient comparison. Magn Reson Med 2011;65:1407-15. [PubMed]
- Jerosch-Herold M, Sheridan DC, Kushner JD, et al. Cardiac magnetic resonance imaging of myocardial contrast uptake and blood flow in patients affected with idiopathic or familial dilated cardiomyopathy. Am J Physiol Heart Circ Physiol 2008;295:H1234-H1242. [PubMed]
- Flett AS, Hayward MP, Ashworth MT, et al. Equilibrium contrast cardiovascular magnetic resonance for the measurement of diffuse myocardial fibrosis: preliminary validation in humans. Circulation 2010;122:138-44. [PubMed]
- White SK, Sado DM, Fontana M, et al. T1 Mapping for myocardial extracellular volume measurement by CMR: Bolus only versus primed infusion technique. JACC Cardiovasc Imaging 2013;6:955-62. [PubMed]
- Pardo Mindán FJ, Panizo A. Alterations in the extracellular matrix of the myocardium in essential hypertension. Eur Heart J 1993;14 Suppl J:12-4.
- Shimizu M, Umeda K, Sugihara N, et al. Collagen remodelling in myocardia of patients with diabetes. J Clin Pathol 1993;46:32-6. [PubMed]
- Westermann D, Lindner D, Kasner M, et al. Cardiac inflammation contributes to changes in the extracellular matrix in patients with heart failure and normal ejection fraction. Circ Heart Fail 2011;4:44-52. [PubMed]
- Sciarretta S, Paneni F, Palano F, et al. Role of the renin-angiotensin-aldosterone system and inflammatory processes in the development and progression of diastolic dysfunction. Clin Sci (Lond) 2009;116:467-77. [PubMed]
- Weber KT, Brilla CG. Pathological hypertrophy and cardiac interstitium. Fibrosis and renin-angiotensin-aldosterone system. Circulation 1991;83:1849-65. [PubMed]
- Iles L, Pfluger H, Phrommintikul A, et al. Evaluation of diffuse myocardial fibrosis in heart failure with cardiac magnetic resonance contrast-enhanced t1 mapping. J Am Coll Cardiol 2008;52:1574-80. [PubMed]
- Sibley CT, Noureldin RA, Gai N, et al. T1 mapping in cardiomyopathy at cardiac mr: Comparison with endomyocardial biopsy. Radiology 2012;265:724-32. [PubMed]
- Fontana M, White SK, Banypersad SM, et al. Comparison of T1 mapping techniques for ecv quantification. Histological validation and reproducibility of shmolli versus multibreath-hold T1 quantification equilibrium contrast cmr. J Cardiovasc Magn Reson 2012;14:88. [PubMed]
- Bull S, White SK, Piechnik SK, et al. Human non-contrast T1 values and correlation with histology in diffuse fibrosis. Heart 2013;99:932-7. [PubMed]
- Karamitsos TD, Piechnik SK, Banypersad SM, et al. Noncontrast T1 mapping for the diagnosis of cardiac amyloidosis. JACC Cardiovasc Imaging 2013;6:488-97. [PubMed]
- Sado DM, White SK, Piechnik SK, et al. Identification and assessment of anderson-fabry disease by cardiovascular magnetic resonance noncontrast myocardial t1 mapping. Circ Cardiovasc Imaging 2013;6:392-8. [PubMed]
- Dass S, Suttie JJ, Piechnik SK, et al. Myocardial tissue characterization using magnetic resonance noncontrast T1 mapping in hypertrophic and dilated cardiomyopathy. Circ Cardiovasc Imaging 2012;5:726-33. [PubMed]
- Amano Y, Takayama M, Kumita S. Contrast-enhanced myocardial T1-weighted scout (look-locker) imaging for the detection of myocardial damages in hypertrophic cardiomyopathy. J Magn Reson Imaging 2009;30:778-84. [PubMed]
- Turkbey EB, Gai N, Lima JA, et al. Assessment of cardiac involvement in myotonic muscular dystrophy by T1 mapping on magnetic resonance imaging. Heart Rhythm 2012;9:1691-7. [PubMed]
- Yingchoncharoen T, Negishi T, Negishi K, et al. Focal fibrosis and diffuse fibrosis are predictors of positive left ventricular remodeling in patients with non-ischemic cardiomyopathy. Circulation 2013;128:A18189.
- Beinart R, Khurram IM, Liu S, et al. Cardiac magnetic resonance T1 mapping of left atrial myocardium. Heart Rhythm 2013;10:1325-31. [PubMed]
- Brooks J, Kramer CM, Salerno M. Markedly increased volume of distribution of gadolinium in cardiac amyloidosis demonstrated by T1 mapping. J Magn Reson Imaging 2013;38:1591-5. [PubMed]
- Puntmann VO, Voigt T, Chen Z, et al. Native T1 mapping in differentiation of normal myocardium from diffuse disease in hypertrophic and dilated cardiomyopathy. JACC Cardiovasc Imaging 2013;6:475-84. [PubMed]
- Ling LH, Kalman JM, Ellims AH, et al. Diffuse ventricular fibrosis is a late outcome of tachycardia-mediated cardiomyopathy after successful ablation. Circ Arrhythm Electrophysiol 2013;6:697-704. [PubMed]
- Puntmann VO, D’Cruz D, Smith Z, et al. Native myocardial T1 mapping by cardiovascular magnetic resonance imaging in subclinical cardiomyopathy in patients with systemic lupus erythematosus. Circ Cardiovasc Imaging 2013;6:295-301. [PubMed]
- Thompson RB, Chow K, Khan A, et al. T1 mapping with cardiovascular MRI is highly sensitive for fabry disease independent of hypertrophy and sex. Circ Cardiovasc Imaging 2013;6:637-45. [PubMed]
- Tham EB, Haykowsky MJ, Chow K, et al. Diffuse myocardial fibrosis by T1-mapping in children with subclinical anthracycline cardiotoxicity: relationship to exercise capacity, cumulative dose and remodeling. J Cardiovasc Magn Reson 2013;15:48. [PubMed]
- Miller CA, Naish JH, Bishop P, et al. Comprehensive validation of cardiovascular magnetic resonance techniques for the assessment of myocardial extracellular volume. Circ Cardiovasc Imaging 2013;6:373-83. [PubMed]
- Klein C, Nekolla SG, Balbach T, et al. The influence of myocardial blood flow and volume of distribution on late Gd-DTPA kinetics in ischemic heart failure. J Magn Reson Imaging 2004;20:588-93. [PubMed]
- Neilan TG, Coelho-Filho OR, Shah RV, et al. Myocardial extracellular volume fraction from T1 measurements in healthy volunteers and mice: relationship to aging and cardiac dimensions. JACC Cardiovasc Imaging 2013;6:672-83. [PubMed]
- Rao AD, Shah RV, Garg R, et al. Aldosterone and myocardial extracellular matrix expansion in type 2 diabetes mellitus. Am J Cardiol 2013;112:73-8. [PubMed]
- Ho CY, Abbasi SA, Neilan TG, et al. T1 measurements identify extracellular volume expansion in hypertrophic cardiomyopathy sarcomere mutation carriers with and without left ventricular hypertrophy. Circ Cardiovasc Imaging 2013;6:415-22. [PubMed]
- Mongeon FP, Jerosch-Herold M, Coelho-Filho OR, et al. Quantification of extracellular matrix expansion by cmr in infiltrative heart disease. JACC Cardiovasc Imaging 2012;5:897-907. [PubMed]
- Ng AC, Auger D, Delgado V, et al. Association between diffuse myocardial fibrosis by cardiac magnetic resonance contrast-enhanced T1 mapping and subclinical myocardial dysfunction in diabetic patients: a pilot study. Circ Cardiovasc Imaging 2012;5:51-9. [PubMed]
- Broberg CS, Chugh SS, Conklin C, et al. Quantification of diffuse myocardial fibrosis and its association with myocardial dysfunction in congenital heart disease. Circ Cardiovasc Imaging 2010;3:727-34. [PubMed]
- Wong TC, Piehler K, Meier CG, et al. Association between extracellular matrix expansion quantified by cardiovascular magnetic resonance and short-term mortality. Circulation 2012;126:1206-16. [PubMed]
- Liu S, Han J, Nacif MS, et al. Diffuse myocardial fibrosis evaluation using cardiac magnetic resonance T1 mapping: sample size considerations for clinical trials. J Cardiovasc Magn Reson 2012;14:90. [PubMed]
- Liu CY, Liu YC, Wu C, et al. Evaluation of age related interstitial myocardial fibrosis with cardiac magnetic resonance contrast-enhanced T1 mapping: MESA (Multi-Ethnic Study of Atherosclerosis. J Am Coll Cardiol 2013;62:1280-7. [PubMed]
- Robbers LF, Baars EN, Brouwer WP, et al. T1 mapping shows increased extracellular matrix size in the myocardium due to amyloid depositions. Circ Cardiovasc Imaging 2012;5:423-6. [PubMed]
- Todiere G, Aquaro GD, Piaggi P, et al. Progression of myocardial fibrosis assessed with cardiac magnetic resonance in hypertrophic cardiomyopathy. J Am Coll Cardiol 2012;60:922-9. [PubMed]
- Ferreira VM, Piechnik SK, Dall’Armellina E, et al. Non-contrast T1-mapping detects acute myocardial edema with high diagnostic accuracy: a comparison to T2-weighted cardiovascular magnetic resonance. J Cardiovasc Magn Reson 2012;14:42. [PubMed]
- Friedrich MG. Myocardial edema--a new clinical entity? Nat Rev Cardiol 2010;7:292-6. [PubMed]
- Dall’Armellina E, Piechnik SK, Ferreira VM, et al. Cardiovascular magnetic resonance by non contrast T1-mapping allows assessment of severity of injury in acute myocardial infarction. J Cardiovasc Magn Reson 2012;14:15. [PubMed]
- Sparrow P, Messroghli DR, Reid S, et al. Myocardial T1 mapping for detection of left ventricular myocardial fibrosis in chronic aortic regurgitation: pilot study. AJR Am J Roentgenol 2006;187:W630-5. [PubMed]
- Weidemann F, Herrmann S, Stork S, et al. Impact of myocardial fibrosis in patients with symptomatic severe aortic stenosis. Circulation 2009;120:577-84. [PubMed]
- Mewton N, Liu CY, Croisille P, et al. Assessment of myocardial fibrosis with cardiovascular magnetic resonance. J Am Coll Cardiol 2011;57:891-903. [PubMed]
- Raman FS, Kawel-Boehm N, Gai N, et al. Modified look-locker inversion recovery T1 mapping indices: assessment of accuracy and reproducibility between magnetic resonance scanners. J Cardiovasc Magn Reson 2013;15:64. [PubMed]
- Piechnik SK, Ferreira VM, Lewandowski AJ, et al. Normal variation of magnetic resonance T1 relaxation times in the human population at 1.5 T using ShMOLLI. J Cardiovasc Magn Reson 2013;15:13. [PubMed]