Non-invasive investigation of myocardial energetics in cardiac disease using 31P magnetic resonance spectroscopy
Introduction
Cardiac metabolism and function are intrinsically linked. High-energy phosphates occupy a central and obligate position in cardiac metabolism, coupling oxygen and substrate fuel delivery to the myocardium with contractile work. This insight underlies the widespread use of stress echocardiography to document inducible wall motion deficits and interpret these as ischaemia: the ischaemic cascade begins with a metabolic perturbation, climbs through diastolic dysfunction, inducible regional wall motion abnormality, and culminates in electrocardiographic changes and symptoms. However, inducible ischaemia is not the only metabolic problem that may or may not be phenotyped in the heart with impaired contractile reserve. Other deficits in high-energy phosphate metabolism (not secondary to supply-demand mismatch of oxygen and substrate fuels) can be documented, and are of particular interest when found in the context of structural heart disease.
This review introduces the scope of deficits in high-energy phosphate metabolism that may be observed in the myocardium, how to assess for them, and how they might be interpreted.
The significance of high-energy phosphates in myocardial work and energy homeostasis
A high-energy phosphate molecule carries a phosphoryl bond (~P) with a large energy of hydrolysis. Reactions that add water and split off the phosphoryl group release inorganic phosphate (Pi) and lots of energy (J/mol). These exergonic reactions (negative free energy, or delta G) are coupled in living cells to molecular motors which require energy to do work. The most important molecular motors in the heart include sarcolemmal Na+-K+-ATPase, sarcoplasmic reticulum Ca2+-ATPase, and myosin-ATPase. Each carries a thermodynamic requirement for a work cycle to proceed spontaneously (i.e., the sum of the negative delta G of ~P hydrolysis and the positive delta G of the work-performing enzyme must be less than zero). Without ~P availability, the cell cannot do work or maintain ionic homeostasis as ~P hydrolysis is obligate for both. This is also referred to as contractile coupling:
Cardioventilatory coupling → Oxygen delivery → ~P → Sarcomere shortening → External work.
The predominant high-energy phosphate is ATP, which has two ~P bonds. ATP hydrolysis results in ADP + Pi. While ADP could be hydrolysed to AMP, and AMP to adenosine, in practice cells try to avoid this as adenosine is lost easily from cells by diffusion and ATP is resource-intensive to synthesise de novo (requiring nine ATPs) (1). Cells also try to maintain very high [ATP]/[ADP][AMP] ratios (phosphorylation potentials) near work-performing enzymes. A first reason for this is that the free energy of ATP hydrolysis is proportional to the [ATP]/[ADP][Pi] ratio.
| [1] |
A second reason is that high cytosolic [ADP] antagonises myosin-actin cross-bridge cycling, and raises the left ventricular end-diastolic pressure-volume slope (2,3). Third, [AMP] is a marker of metabolic stress, and directly activates AMP-activated protein kinase (AMPK). AMPK is an evolutionarily-conserved enzyme that acts as a sensor of metabolic stress and responds by activating ATP-supplying catabolic pathways and suppressing ATP-requiring anabolic pathways (4,5). Fourth, Pi is a direct stimulant of glycogenolysis and anaerobic glycolysis (at the level of phosphofructokinase). Glycogen is the only carbohydrate store in cardiomyocytes and is metabolically costly to synthesise and to maintain (attracting much more water mass than lipid per unit energy stored).
In practice therefore, several mechanisms have evolved to maintain high-energy phosphate metabolic (i.e., energetic) homeostasis – high [ATP]/[ADP][AMP] ratios and low [Pi] in the cytosol. Conversely, metabolic stressors, whether they be exercise, ischaemia, pressure-overload, or glucose-deprivation, may or may not perturb this energetic signature depending on their severity, duration and the existing metabolic reserve.
The first defence against metabolic stressors a high-energy phosphate-donor (phosphagen) system, which in vertebrate muscle is the creatine kinase (CK) system (6). CK buffers ATP concentrations in cells during fluctuations in energy demand. The CK system comprises a phosphate donor (phosphocreatine, PCr), a phosphate acceptor (free creatine, Cr), and a catalytic enzyme (CK).
| [2] |
The phosphagen PCr was the first recognised high-energy phosphate (7), and is enriched in cardiac muscle leading to a PCr/ATP ratio of around 2:1 at baseline. The reversible transfer of ~P from PCr to ADP (‘phosphotransfer’), established in 1935 (8), effectively triples the ~P reserve that the cardiomyocyte will willingly expend before requiring more ATP resynthesis (from fuel and oxygen) or the hydrolysis of ADP or AMP. CK enzymes are also enriched in muscle. CK isoenzymes may be cytosolic, where they exist primarily as homodimers (CK-MM is the predominant form in adult hearts, with CK-MB and -BB much less common), or mitochondrial, where they exist primarily as octamers (mitochondrial-CK). Cytosolic isoenzymes primarily catalyse the forward, or ATP-producing direction of the equilibrium reaction, while mitochondrial isoenzymes catalyse the PCr-producing direction.
A second line of defence is adenylate kinase, which catalyses the equilibrium 2ADP ↔ ATP + AMP. This is of minor importance in the healthy heart, where it accounts for around 10% of total phosphotransfer (9), but becomes more important in ischaemia, where it effectively allows a second ~P to be used from ATP, and in animal heart failure models, where it can take on more of total cellular phosphotransfer to compensate for any reduction in total CK activity.
Third and fourth lines of defence have already been mentioned: AMPK activation and activation of anaerobic carbohydrate catabolism.
A less immediate form of defence is cytoarchitectural remodelling to reduce the diffusion time for ATP and ADP between mitochondria and myofibrils. ATP may be delivered to (and ADP removed from) sites of use by simple diffusion (10) and facilitated diffusion (the CK system) (11). Although there is disagreement over the relative importance of these processes in cardiac muscle (12), a reduction in diffusion distances between mitochondria and myofibrils has been observed in M-CK/mitochondrial-CK double knockout mouse myocardium (13). The promotion of “direct channelling” of adenine nucleotides between organelles in the setting of reduced CK activity may underlie the preservation of cardiac function under moderate workloads in these hearts [reviewed (14)].
In summary, ATP occupies a central and obligate position in cardioventilatory coupling to external work. Changes in ATP homeostasis should be interpreted in the context of the prevailing metabolic stress and may indicate problems with metabolic reserve. Observed deficits in high-energy phosphate metabolism may include any or all of: reductions in [ATP][PCr]/[ADP][Cr], total CK activity, and rises in [Pi] and [AMP].
Techniques for interrogating myocardial high-energy phosphate metabolism
From 1927-77, biochemical analysis of rapidly flash-frozen myocardial biopsies was the only method to interrogate energetic homeostasis. This remains the gold standard for assessing total CK activity, total creatine concentration (PCr + Cr), and total adenine nucleotides (ATP + ADP + AMP). However, the lability of PCr and ATP means that PCr, ADP, and Pi cannot be reliably quantified using techniques inherently destructive of the tissue under study [ATP comprises ~85% of total adenine nucleotides (2)].
31Phosphorus (31P)-magnetic resonance spectroscopy (MRS) is a powerful and non-invasive technique to assess cellular energetics and is complementary to biochemical analysis. All magnetic resonance techniques include two fundamental steps: firstly excitation of the nuclei of interest using radiofrequency (RF) energy, and secondly the recording of induced current in the receiver coils of the scanner after the RF energy is switched off. The amplitude of the signal received is proportional to the number of nuclei within the interrogated volume of tissue. In MRS, the signal induced in the receiver coils by the relaxing and dephasing nuclei, or ‘free induction decay’, is simplified by transforming it to the frequency domain (Figure 1).
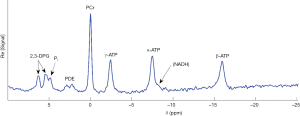
A 31P spectrum is created by plotting signal amplitude against ‘chemical shift’ (δ), that is, resonant frequency (ν) relative to a reference frequency (νref). The units of chemical shift are parts per million, ppm:
| [3] |
Chemical shift is field strength-independent and arises because different nuclei are shielded by nearby electrons to different extents. This shielding causes the effective field strength, and thus resonant frequency, to vary slightly between nuclei. For example, the 1H spectrum of a volume of pure water should have a single peak, as all protons experience equivalent electron shielding. However, the 1H spectrum of a volume of pure ethanol (CH3CH2OH) has three peaks, with amplitudes in a 3:2:1 ratio, corresponding to the CH3-, CH2-, and -OH environments.
At clinical field strengths [1.5 and 3 Tesla (T)], the resonant frequencies of 1H, 13C, 23Na, 31P are widely separated and these nuclei cannot be co-excited. However, the resonant frequencies of moeities containing 31P nuclei are much closer together—within 25 ppm of each other. Plotting the signal amplitudes against their chemical shifts relative to a designated reference nucleus (PCr) results in six visible peaks at 3T: 2,3-DPG/Pi, PDE, PCr, and three ATP peaks (Figure 1).
At 3T, a cardiac 31P spectrum can be obtained from a 3D-localised myocardial voxel (nominal size 5.6 mL) in 11 minutes at our institution (16). In practice, 20 minutes are required if preparatory steps are included. A longer acquisition time would be required if smaller voxels or greater signal-noise-ratio was desired. Such spectra are most commonly used to estimate the PCr/ATP ratio as a key index of energetic state, but in theory could also be used to calculate absolute metabolite concentrations, intracellular [Mg] (from the shift of β-ATP relative to PCr), and pH (from the shift of Pi relative to PCr) (17). While signal-noise ratio improves linearly with field strength, these gains are only realised if increases in field strength inhomogeneity, RF energy deposition, RF thermal noise and RF energy requirements are handled acceptably (18). The potential of 31P-MRS at 7T probably lies in the quantification of [Pi], a molecule that is normally obscured by the overlapping blood 2,3-DPG signal and/or too small (low in concentration) to be reliably detectable.
The key advantages of 31P-MRS over biochemical techniques for the assessment of cardiac energetic state are firstly that it is non-destructive (allowing repeat assessments over time and correlation with contractile function), and secondly that it can assess the labile PCr and Pi molecules. As a result, 31P-MRS has been used to study the effects of acute changes in blood flow (19-22), workload (23), substrate availability, and to assess CK activity (2,3,24,25).
The key limitation of 31P-MRS is an inherently low signal-noise ratio (roughly 105-fold reduced compared with 1H-MRS). Spectral quality can be improved by using surface coils placed as close as possible to the myocardium, although techniques to minimise contamination by skeletal wall muscle are also required. 31P-MRS is relatively insensitive to metabolites at low concentrations (< ~0.5 mM), and long acquisition times. In addition, current use of many surface coils results in relative insensitivity to the posterior myocardial wall due to the greater distance from the coil. 31P-MRS is therefore better suited currently to studying global rather than regional myocardial pathologies, although technical work continues to achieve whole-heart excitation.
The respective intracellular concentrations of PCr, free creatine, ATP, ADP, AMP, and Pi are on the order of 24, 16, 12, 0.04, 1, and 0.1 mM (1,26,27). In particular, the difficult metabolites are: Pi (overlapped by 2,3-DPG), β-ADP and α-ADP (whose chemical shifts overlap those of γ-ATP and α-ATP respectively), and AMP (a phosphomonoester which lies downfield of (i.e., to the left of) Pi on a 31P spectrum (Figure 1) and is overlapped by glucose-6-phosphate, phosphocholine and phosphoethanolamine). 31P-MRS is also insensitive to non-mobile metabolites (as fixed nuclei have shorter spin-spin relaxation times (T2s), and spectral line-width is proportional to 1/T2), but in practice this is helpful as it silences signal from DNA- and macromolecule-bound nucleoside triphosphates.
In addition to acquiring snapshots of relative metabolite concentrations at single timepoints, 31P-MRS can also be used to interrogate dynamic reaction kinetics. In its simplest form, this can be performed by acquiring sequential 31P spectra before and after a metabolic perturbation such as exercise. Analysis of energetic and pH recovery rates can enable estimation of ATP synthesis rates (by oxidative phosphorylation and/or CK) though to date, such studies in humans have been restricted to skeletal muscle (17).
A second dynamic 31P-MRS technique employs magnetisation saturation transfer. Saturation transfer techniques involve introducing a magnetic resonance label (i.e., a non-equilibrium nuclear spin magnetisation) onto a molecule of interest using selective RF pulses. If the chemical exchange is rapid enough, this magnetic label transfers to other moieties before it is relaxed by the spin-lattice (T1) mechanism. By observing this transfer, the rate constants for chemical exchange can be determined. These rate constants are unusual as they are unidirectional and first-order, even though the underlying reaction may not be.
Two broad classes of magnetisation transfer experiment include the steady state experiment, where the label is continuously introduced and signal is recorded after the system comes to a new steady state, and the temporal experiment, where the label is introduced transiently. The principles underlying these experiments have been reviewed elsewhere (28-31). The only reaction studied to date in human myocardium is the CK reaction in the ATP-producing direction, which requires continuous selective saturation of the γ-ATP resonance. Human studies date from 2002, and have employed four sequences at two centres: four-angle saturation transfer at 1.5T (32) and 7T (33), and triple repetition time saturation transfer (15,34,35) and two repetition time saturation transfer (36), both at 3T. All measure the unidirectional forward rate constant of CK, kf, which when multiplied by substrate concentration (kf × [PCr]) gives total (not net) unidirectional forward flux. The reverse CK reaction could in principle be studied, as could ATP hydrolysis, but in practice the requirement to concomitantly and selectively saturate Pi remains limiting.
A third technique, 18O-assisted 31P-MRS (37,38) is able to infer net reaction rates by following the rate of incorporation of 18O (provided from 18O-labelled water) to Pi (ATP hydrolysis), to γ-ATP (ATP synthesis), and then to either PCr (CK), β-ADP and β-ATP (adenylate kinase) or glucose-6-phosphate (hexokinase). Its key disadvantage is that it requires mass spectrometry i.e., ex vivo tissue samples.
In summary, the above techniques collectively allow interrogation of cardiac total creatine, total CK activity, and total adenine nucleotide pool (biopsy), and of PCr, ATP, Pi, pH, CK kf, and CK total unidirectional flux in either direction (31P-MRS). The remaining moieties desirable to quantify are ADP and free creatine. ADP is usually calculated by rearranging the equation for the CK equilibrium constant, with the proviso that this extrapolates from a constant established in vitro for rat CK (39,40). Free creatine may be calculated by subtracting absolute [PCr] determined non-invasively (using a within-study tissue calibration) from total creatine by biopsy; it may also be non-invasively estimated by 1H-MRS.
Deficits in high-energy phosphate metabolism that may be observed in the myocardium
Human cardiac 31P-MRS studies have documented reductions in myocardial PCr/ATP ratio in established non-ischaemic cardiomyopathy (41-43), heart failure with preserved ejection fraction (44), hypertrophic cardiomyopathy (45,46), hypertensive hypertrophy with or without heart failure (47,48), severe aortic stenosis with or without heart failure (49-51), moderate aortic stenosis with preserved left ventricular systolic function (author’s data, under review), severe primary mitral regurgitation in patients with an indication for operative repair [(52) and author’s own data], short term high fat diet (53), obese volunteers (54,55), and patients with insulin resistance (56) or diabetes mellitus but no known heart disease (57-61). There is also a weak negative correlation with age (62-65).
A reduction in the PCr/ATP ratio is therefore an energetic signature common to numerous conditions which can predispose to heart failure, and this finding is supported by studies in animal models. Furthermore, a reduction in the PCr/ATP ratio predicts prognosis in non-ischaemic cardiomyopathy (66), and may improve with heart failure treatment (41), after aortic valve replacement (51,67), after weight loss (68), or with trimetazidine in heart failure (69), suggesting that reduced PCr/ATP ratio is not necessarily simply an age-related phenomenon, and that energetics may be central to disease pathogenesis.
Several studies have also documented further reductions in PCr/ATP in the face of acute metabolic stress, most commonly using sustained catecholamine infusions at moderate dose (54,59,70). However, not all studies in diabetes mellitus (71) or heart failure document reduced PCr/ATP ratio at rest (72-75) or during dobutamine stress (76)—this may partly reflect a pseudo-normalisation of the resting ratio in late disease when absolute ATP concentrations reduce, and may also reflect differences in body weight, disease stage or degree of stress.
The above references are only a selection of studies performed; there is a body of animal and human work not discussed here which complements non-invasive phenotyping with biopsy assessments of total CK activity, CK isoform composition, total creatine, and/or total adenine nucleotide pool, or assesses metabolic factors upstream of ~P generation (substrate handling, mitochondrial capacity, oxidative phosphorylation). For this, the reader is referred to further reviews (77-86).
The underlying mechanism for a reduction in PCr/ATP ratio is not firmly established in all cases, but two explanations have merit in myocardial hypertrophy secondary to chronically increased work. Firstly, there may be increases in ATP consumption rate per gram of myocardium, so shifting the CK equilibrium to the right (toward ATP) in the presence of limited phosphotransfer reserve. Secondly, there may be a fall in cardiomyocyte total creatine concentration. The cell may then respond to this by shifting the equilibrium to the right, prioritising the ATP/ADP ratio at the cost of the PCr/free creatine ratio. Underlying this there may be changes in the CK equilibrium constant, which has not been assessed in human muscle or in different disease states (39,40).
Two more recently described variables in human myocardium are CK kf and CK total unidirectional forward flux. Flux is arguably a more important variable than metabolite pool size (PCr, ATP etc) as it correlates more closely with contractile reserve (23,87-89), post-ischaemic functional recovery (37,90), and end-diastolic wall tension (2,3,24). Greater flux dampens projected beat-to-beat fluctuations in metabolite pool sizes, particularly of ATP and ADP [see (91) for assumptions implicit to in silico models]. Unidirectional forward flux was reduced in human hypertensive hypertrophy with failure (48), non-ischaemic cardiomyopathy (72,92), and ischaemic cardiomyopathy (73), and this reduction in CK forward flux correlated with non-invasively estimated cardiac work (93) and carried prognostic value (72). These data are consistent with biopsy studies documenting reductions in total CK activity in human non-ischaemic cardiomyopathy (94-97) and severe aortic stenosis with or without heart failure [(98) and author’s own data, under review], and with within-patient correlations of global circumferential strain by MRI, LV ejection fraction, and LV end-systolic volume index against biopsy-determined total CK velocity in patients with severe aortic stenosis (author’s data, under review).
Quantifying [Pi] reliably is an important goal in 31P-MRS research because [Pi] is required to estimate the free energy of ATP hydrolysis. It is also important kinetically, both limiting the rate of ATP hydrolysis (99) and stimulating oxidative phosphorylation (100), and at the level of substrate selection, stimulating glycogen catabolism and glycolysis. Splitting (and growth) of the Pi peak to indicate two compartments, each with different pH, is a classic indicator of acute ischaemia (101-104). In human myocardium a few groups have described the Pi/PCr ratio: in hypertrophic cardiomyopathy (75,105-107) and in non-ischaemic cardiomyopathy and healthy volunteers with and without dobutamine stress (unpublished). However, reliable signal from non-hypertrophied myocardium is only obtainable at the present time at 7T and further technical development is required.
The meaningfulness of these mostly small cross-sectional studies is somewhat paradoxical. On the one hand they afford a unique insight into ~P, a central and obligate step linking metabolic and contractile reserve. The recent observation of an increase in resting myocardial kf and reduced responsiveness of kf to acute dobutamine stress in obese volunteers, with reversal of the energetic phenotype after weight loss (55) raises intriguing questions about whether energetic improvement may underlie the reduction in atrial fibrillation burden also observed with weight loss (108). On the other hand, longitudinal studies of energetic phenotype controlling for covariates with known prognostic impact are lacking in a contemporary heart failure (or at-risk) cohort. Empa-Vision (NCT03332212), a longitudinal study of the effect of empaglifozin on energetic and contractile phenotype in non-ischaemic heart failure, is an example of the kind of study required to address this. The effect of cardiotoxic chemotherapy upon myocardial energetics is also relatively unstudied.
The relevance of a depressed metabolic phenotype to clinical management remains an open question, as it is not known to what extent an abnormal energetic phenotype permits or maintains the heart failure syndrome, versus simply being a bystander consequence (109). While upregulation of certain elements of the CK system can be cardioprotective [e.g., CK isozymes (110-113), total creatine (114,115)] and knockout (116) or inhibition (117) of certain elements can be detrimental, the same can be said for many parallel processes within the cardiomyocyte and do not resolve the question. Rather, they illustrate the consequence of extremes of energetic deficiency. Clinical trials of interventions that increase CK activity (118) or normalise energetic phenotypes (deliberately or not) are a required but as yet missing piece to this puzzle.
Conclusions
31P-MRS affords a unique window into high-energy phosphate metabolism, which is an obligate step linking myocardial oxygen and fuel delivery with contractile work. Most work to date has focussed on the PCr/ATP ratio in various preclinical and disease states, but further work is required to understand not just whether it is depressed, but why, and to explore CK kf and Pi more thoroughly. Together with better 1H-MRS assessment of cardiac total creatine, non-invasive assessment of the amount of energy available from ATP would then become within reach. Ultimately, longitudinal studies assessing the effects of myocardial therapies on contractile phenotype, energetic phenotype, and clinical events (prognosis) will be required to assess to what extent energetic dysfunction is a bystander versus an active problem in myocardial dysfunction and heart failure.
Acknowledgments
Funding: None.
Footnote
Provenance and Peer Review: This article was commissioned by the Guest Editors (Oliver Rider and Andrew J. Lewis) for the series “The use of advanced cardiac MRI in heart failure and cardiac hypertrophy” published in Cardiovascular Diagnosis and Therapy. The article was sent for external peer review organized by the Guest Editors and the editorial office.
Conflicts of Interests: All authors have completed the ICMJE uniform disclosure form (available at http://dx.doi.org/10.21037/cdt-20-275). The series “The use of advanced cardiac MRI in heart failure and cardiac hypertrophy” was commissioned by the editorial office without any funding or sponsorship. AJML and OJR served as the unpaid Guest Editors of the series. AJML reports other from Bayer, personal fees from Boehringer Ingelheim, outside the submitted work. SN reports grants from British Heart Foundation, during the conduct of the study; grants from Cytokinetics, personal fees from Cytokinetics, personal fees from Pfizer, grants from Boehringer Ingelheim, outside the submitted work. MAP reports grants from British Heart Foundation No. FS/15/80/31803, outside the submitted work. The other authors have no other conflicts of interest to declare.
Ethical statement: The authors are accountable for all aspects of the work in ensuring that questions related to the accuracy or integrity of any part of the work are appropriately investigated and resolved.
Open Access Statement: This is an Open Access article distributed in accordance with the Creative Commons Attribution-NonCommercial-NoDerivs 4.0 International License (CC BY-NC-ND 4.0), which permits the non-commercial replication and distribution of the article with the strict proviso that no changes or edits are made and the original work is properly cited (including links to both the formal publication through the relevant DOI and the license). See: https://creativecommons.org/licenses/by-nc-nd/4.0/.
References
- Ingwall JS. ATP and the Heart. Springer US, 2002.
- Tian R, Christe ME, Spindler M, et al. Role of MgADP in the development of diastolic dysfunction in the intact beating rat heart. J Clin Invest 1997;99:745-51. [Crossref] [PubMed]
- Tian R, Nascimben L, Ingwall JS, et al. Failure to maintain a low ADP concentration impairs diastolic function in hypertrophied rat hearts. Circulation 1997;96:1313-9. [Crossref] [PubMed]
- Hardie DG. AMP-activated protein kinase: maintaining energy homeostasis at the cellular and whole-body levels. Annu Rev Nutr 2014;34:31-55. [Crossref] [PubMed]
- Hardie DG. AMPK - Sensing Energy while Talking to Other Signaling Pathways. Cell Metab 2014;20:939-52. [Crossref] [PubMed]
- Wallimann T, Wyss M, Brdiczka D, et al. Intracellular compartmentation, structure and function of creatine kinase isoenzymes in tissues with high and fluctuating energy demands: the 'phosphocreatine circuit' for cellular energy homeostasis. Biochem J 1992;281:21-40. [Crossref] [PubMed]
- Fiske CH, Subbarow Y. The nature of the "inorganic phosphate" in voluntary muscle. Science 1927;65:401-3. [Crossref] [PubMed]
- Lehmann H. Über die enzymatische Synthese der Kreatinphosphorsäure durch Umesterung der Phosphobrenztraubensäure. Biochem Z 1935;281:271-91.
- Dzeja PP, Vitkevicius KT, Redfield MM, et al. Adenylate Kinase-Catalyzed Phosphotransfer in the Myocardium: Increased Contribution in Heart Failure. Circ Res 1999;84:1137-43. [Crossref] [PubMed]
- Jacobus WE. Theoretical support for the heart phosphocreatine energy transport shuttle based on the intracellular diffusion limited mobility of ADP. Biochem Biophys Res Commun 1985;133:1035-41. [Crossref] [PubMed]
- Meyer RA, Sweeney HL, Kushmerick MJ. A simple analysis of the "phosphocreatine shuttle". Am J Physiol 1984;246:C365-77. [Crossref] [PubMed]
- Greenhaff PL. The creatine-phosphocreatine system: there's more than one song in its repertoire. J Physiol 2001;537:657. [Crossref] [PubMed]
- Kaasik A, Veksler V, Boehm E, et al. Energetic Crosstalk Between Organelles: Architectural Integration of Energy Production and Utilization. Circ Res 2001;89:153-9. [Crossref] [PubMed]
- Ventura-Clapier R, Kaasik A, Veksler V. Structural and functional adaptations of striated muscles to CK deficiency. Mol Cell Biochem 2004;256-257:29-41. [Crossref] [PubMed]
- Clarke WT. Human Cardiac Magnetic Resonance Spectroscopy. University of Oxford; 2016. p. 320.
- Tyler DJ, Hudsmith LE, Clarke K, et al. A comparison of cardiac 31P MRS at 1.5 and 3 T. NMR Biomed 2008;21:793-8. [Crossref] [PubMed]
- Valkovič L, Chmelík M, Krššák M. In-vivo 31P-MRS of skeletal muscle and liver: A way for non-invasive assessment of their metabolism. Anal Biochem 2017;529:193-215. [Crossref] [PubMed]
- Rodgers CT, Clarke WT, Snyder C, et al. Human cardiac 31P magnetic resonance spectroscopy at 7 tesla. Magn Reson Med 2014;72:304-15. [Crossref] [PubMed]
- Gudbjarnason S, Mathes P, Ravens KG. Functional compartmentation of ATP and creatine phosphate in heart muscle. J Mol Cell Cardiol 1970;1:325-39. [Crossref] [PubMed]
- Bittl JA, Weisfeldt ML, Jacobus WE. Creatine kinase of heart mitochondria. The progressive loss of enzyme activity during in vivo ischemia and its correlation to depressed myocardial function. J Biol Chem 1985;260:208-14. [PubMed]
- Bittl JA, Balschi JA, Ingwall JS. Contractile failure and high-energy phosphate turnover during hypoxia: 31P-NMR surface coil studies in living rat. Circ Res 1987;60:871-8. [Crossref] [PubMed]
- Neubauer S, Hamman BL, Perry SB, et al. Velocity of the creatine kinase reaction decreases in postischemic myocardium: a 31P-NMR magnetization transfer study of the isolated ferret heart. Circ Res 1988;63:1-15. [Crossref] [PubMed]
- Bittl JA, Ingwall JS. Reaction rates of creatine kinase and ATP synthesis in the isolated rat heart. A 31P NMR magnetization transfer study. J Biol Chem 1985;260:3512-7. [PubMed]
- Harrison GJ, van Wijhe MH, de Groot B, et al. Glycolytic buffering affects cardiac bioenergetic signaling and contractile reserve similar to creatine kinase. Am J Physiol Heart Circ Physiol 2003;285:H883-90. [Crossref] [PubMed]
- Kapelko VI, Lakomkin VL, Korchazhkina OV, et al. Cardiac pump function of the isolated rat heart at two modes of energy deprivation and effect of adrenergic stimulation. Mol Cell Biochem 1996;163-164:131-6. [Crossref] [PubMed]
- Balaban RS. The application of nuclear magnetic resonance to the study of cellular physiology. Am J Physiol 1984;246:C10-9. [Crossref] [PubMed]
- Meyer RA, Kuchmerick MJ, Brown TR. Application of 31P-NMR spectroscopy to the study of striated muscle metabolism. Am J Physiol 1982;242:C1-11. [Crossref] [PubMed]
- Alger JR, Shulman RG. NMR Studies of Enzymatic Rates in vitro and in Vivo by Magnetization Transfer. Q Rev Biophys 1984;17:83-124. [Crossref] [PubMed]
- Brindle KM, Radda GK. Measurements of exchange in the reaction catalysed by creatine kinase using 14C and 15N isotope labels and the NMR technique of saturation transfer. Biochim Biophys Acta 1985;829:188-201. [Crossref] [PubMed]
- Ingwall JS. Phosphorus nuclear magnetic resonance spectroscopy of cardiac and skeletal muscles. Am J Physiol 1982;242:H729-44. [PubMed]
- Bottomley PA. Measuring Biochemical Reaction Rates In Vivo with Magnetization Transfer. eMagRes 2016;5:843-58.
- Bottomley PA, Ouwerkerk R, Lee RF, et al. Four-angle saturation transfer (FAST) method for measuring creatine kinase reaction rates in vivo. Magn Reson Med 2002;47:850-63. [Crossref] [PubMed]
- Clarke WT, Robson MD, Neubauer S, et al. Creatine kinase rate constant in the human heart measured with 3D-localization at 7 tesla. Magn Reson Med 2017;78:20-32. [Crossref] [PubMed]
- Schär M, El-Sharkawy AMM, Weiss RG, et al. Triple repetition time saturation transfer (TRiST) 31P spectroscopy for measuring human creatine kinase reaction kinetics. Magn Reson Med 2010;63:1493-501. [Crossref] [PubMed]
- Clarke WT, Peterzan MA, Rayner JJ, et al. Localized rest and stress human cardiac creatine kinase reaction kinetics at 3 T. NMR Biomed 2019;32:e4085. [Crossref] [PubMed]
- Schär M, Gabr RE, El-Sharkawy AM, et al. Two repetition time saturation transfer (TwiST) with spill-over correction to measure creatine kinase reaction rates in human hearts. J Cardiovasc Magn Reson 2015;17:70. [Crossref] [PubMed]
- Pucar D, Dzeja PP, Bast P, et al. Cellular Energetics in the Preconditioned State: protective role for phosphotransfer reactions captured by 18O-assisted 31P NMR. J Biol Chem 2001;276:44812-9. [Crossref] [PubMed]
- Dzeja PP, Hoyer K, Tian R, et al. Rearrangement of energetic and substrate utilization networks compensate for chronic myocardial creatine kinase deficiency. The J Physiol 2011;589:5193-211. [Crossref] [PubMed]
- Lawson JW, Veech RL. Effects of pH and free Mg2+ on the Keq of the creatine kinase reaction and other phosphate hydrolyses and phosphate transfer reactions. J Biol Chem 1979;254:6528-37. [PubMed]
- Veech RL, Lawson JW, Cornell NW, et al. Cytosolic phosphorylation potential. J Biol Chem 1979;254:6538-47. [PubMed]
- Neubauer S, Krahe T, Schindler R, et al. 31P magnetic resonance spectroscopy in dilated cardiomyopathy and coronary artery disease. Altered cardiac high-energy phosphate metabolism in heart failure. Circulation 1992;86:1810-8. [Crossref] [PubMed]
- Hardy CJ, Weiss RG, Bottomley PA, et al. Altered myocardial high-energy phosphate metabolites in patients with dilated cardiomyopathy. Am Heart J 1991;122:795-801. [Crossref] [PubMed]
- Neubauer S, Horn M, Pabst T, et al. Contributions of 31P-magnetic resonance spectroscopy to the understanding of dilated heart muscle disease. Eur Heart J 1995;16:115-8. [Crossref] [PubMed]
- Mahmod M, Pal N, Rayner J, et al. The interplay between metabolic alterations, diastolic strain rate and exercise capacity in mild heart failure with preserved ejection fraction: A cardiovascular magnetic resonance study. J Cardiovasc Magn Reson 2018;20:88. [Crossref] [PubMed]
- Crilley JG, Boehm EA, Blair E, et al. Hypertrophic cardiomyopathy due to sarcomeric gene mutations is characterized by impaired energy metabolism irrespective of the degree of hypertrophy. J Am Coll Cardiol 2003;41:1776-82. [Crossref] [PubMed]
- Ashrafian H, Redwood C, Blair E, et al. Hypertrophic cardiomyopathy:a paradigm for myocardial energy depletion. Trends Genet 2003;19:263-8. [Crossref] [PubMed]
- Lamb HJ, Beyerbacht HP, van der Laarse A, et al. Diastolic Dysfunction in Hypertensive Heart Disease Is Associated With Altered Myocardial Metabolism. Circulation 1999;99:2261-7. [Crossref] [PubMed]
- Smith CS, Bottomley PA, Schulman SP, et al. Altered Creatine Kinase Adenosine Triphosphate Kinetics in Failing Hypertrophied Human Myocardium. Circulation 2006;114:1151-8. [Crossref] [PubMed]
- Conway MA, Allis J, Ouwerkerk R, et al. Detection of low phosphocreatine to ATP ratio in failing hypertrophied human myocardium by 31P magnetic resonance spectroscopy. Lancet 1991;338:973-6. [Crossref] [PubMed]
- Neubauer S, Horn M, Pabst T, et al. Cardiac high-energy phosphate metabolism in patients with aortic valve disease assessed by 31P-magnetic resonance spectroscopy. J Investig Med 1997;45:453-62. [PubMed]
- Mahmod M, Francis JM, Pal N, et al. Myocardial perfusion and oxygenation are impaired during stress in severe aortic stenosis and correlate with impaired energetics and subclinical left ventricular dysfunction. J Cardiovasc Magn Reson 2014;16:29. [Crossref] [PubMed]
- Conway MA, Bottomley PA, Ouwerkerk R, et al. Mitral Regurgitation: Impaired Systolic Function, Eccentric Hypertrophy, and Increased Severity Are Linked to Lower Phosphocreatine/ATP Ratios in Humans. Circulation 1998;97:1716-23. [Crossref] [PubMed]
- Holloway CJ, Cochlin LE, Emmanuel Y, et al. A high-fat diet impairs cardiac high-energy phosphate metabolism and cognitive function in healthy human subjects. Am J Clin Nutr 2011;93:748-55. [Crossref] [PubMed]
- Rider OJ, Francis JM, Ali MK, et al. Effects of Catecholamine Stress on Diastolic Function and Myocardial Energetics in Obesity. Circulation 2012;125:1511-9. [Crossref] [PubMed]
- Rayner JJ, Peterzan MA, Watson WD, et al. Myocardial Energetics in Obesity: Enhanced ATP Delivery Through Creatine Kinase With Blunted Stress Response. Circulation 2020;141:1152-63. [Crossref] [PubMed]
- Perseghin G, Cobelli FD, Esposito A, et al. Left ventricular function and energy metabolism in middle-aged men undergoing long-lasting sustained aerobic oxidative training. Heart 2009;95:630-5. [Crossref] [PubMed]
- Scheuermann-Freestone M, Madsen PL, Manners D, et al. Abnormal Cardiac and Skeletal Muscle Energy Metabolism in Patients With Type 2 Diabetes. Circulation 2003;107:3040-6. [Crossref] [PubMed]
- Shivu GN, Phan TT, Abozguia K, et al. Relationship between coronary microvascular dysfunction and cardiac energetics impairment in type 1 diabetes mellitus. Circulation 2010;121:1209-15. [Crossref] [PubMed]
- Levelt E, Rodgers CT, Clarke WT, et al. Cardiac energetics, oxygenation, and perfusion during increased workload in patients with type 2 diabetes mellitus. Eur Heart J 2016;37:3461-9. [Crossref] [PubMed]
- Metzler B, Schocke MFH, Steinboeck P, et al. Decreased High-Energy Phosphate Ratios in the Myocardium of Men with Diabetes Mellitus Type I. J Cardiovasc Magn Reson 2002;4:493-502. [Crossref] [PubMed]
- Perseghin G, Fiorina P, De Cobelli F, et al. Cross-sectional assessment of the effect of kidney and kidney-pancreas transplantation on resting left ventricular energy metabolism in type 1 diabetic-uremic patients: a phosphorous-31 magnetic resonance spectroscopy study. J Am Coll Cardiol 2005;46:1085-92. [Crossref] [PubMed]
- Jakovljevic DG, Papakonstantinou L, Blamire AM, et al. Effect of physical activity on age-related changes in cardiac function and performance in women. Circ Cardiovasc Imaging 2014;8:e002086. [PubMed]
- Hollingsworth KG, Blamire AM, Keavney BD, et al. Left ventricular torsion, energetics, and diastolic function in normal human aging. Am J Physiol Heart Circ Physiol 2012;302:H885-H892. [Crossref] [PubMed]
- Esterhammer R, Klug G, Wolf C, et al. Cardiac High-Energy Phosphate Metabolism Alters with Age as Studied in 196 Healthy Males with the Help of 31-Phosphorus 2-Dimensional Chemical Shift Imaging. PLoS ONE 2014;9:e97368. [Crossref] [PubMed]
- Nathania M, Hollingsworth KG, Bates M, et al. Impact of age on the association between cardiac high-energy phosphate metabolism and cardiac power in women. Heart 2018;104:111-8. [Crossref] [PubMed]
- Neubauer S, Horn M, Cramer M, et al. Myocardial Phosphocreatine-to-ATP Ratio Is a Predictor of Mortality in Patients With Dilated Cardiomyopathy. Circulation 1997;96:2190-6. [Crossref] [PubMed]
- Beyerbacht HP, Lamb HJ, van der Laarse A, et al. Aortic Valve Replacement in Patients with Aortic Valve Stenosis Improves Myocardial Metabolism and Diastolic Function. Radiology 2001;219:637-43. [Crossref] [PubMed]
- Rider OJ, Francis JM, Tyler D, et al. Effects of weight loss on myocardial energetics and diastolic function in obesity. Int J Cardiovasc Imaging 2013;29:1043-50. [Crossref] [PubMed]
- Fragasso G, Perseghin G, De Cobelli F, et al. Effects of metabolic modulation by trimetazidine on left ventricular function and phosphocreatine/adenosine triphosphate ratio in patients with heart failure. Eur Heart J 2006;27:942-8. [Crossref] [PubMed]
- Dass S, Cochlin LE, Suttie JJ, et al. Exacerbation of cardiac energetic impairment during exercise in hypertrophic cardiomyopathy: a potential mechanism for diastolic dysfunction. Eur Heart J 2015;36:1547-54. [Crossref] [PubMed]
- Rijzewijk LJ, van der Meer RW, Lamb HJ, et al. Altered myocardial substrate metabolism and decreased diastolic function in nonischemic human diabetic cardiomyopathy: studies with cardiac positron emission tomography and magnetic resonance imaging. J Am Coll Cardiol 2009;54:1524-32. [Crossref] [PubMed]
- Bottomley PA, Panjrath GS, Lai S, et al. Metabolic rates of ATP transfer through creatine kinase (CK Flux) predict clinical heart failure events and death. Sci Transl Med 2013;5:215re3. [Crossref] [PubMed]
- Bottomley PA, Wu KC, Gerstenblith G, et al. Reduced Myocardial Creatine Kinase Flux in Human Myocardial Infarction: An In Vivo Phosphorus Magnetic Resonance Spectroscopy Study. Circulation 2009;119:1918-24. [Crossref] [PubMed]
- Beer M, Seyfarth T, Sandstede J, et al. Absolute concentrations of high-energy phosphate metabolites in normal, hypertrophied, and failing human myocardium measured noninvasively with (31)P-SLOOP magnetic resonance spectroscopy. J Am Coll Cardiol 2002;40:1267-74. [Crossref] [PubMed]
- de Roos A, Doornbos J, Luyten PR, et al. Cardiac metabolism in patients with dilated and hypertrophic cardio-myopathy: Assessment with proton-decoupled P-31 MR spectroscopy. J Magn Reson Imaging 1992;2:711-9. [Crossref] [PubMed]
- Schaefer S, Schwartz GG, Steinman SK, et al. Metabolic response of the human heart to inotropic stimulation: In vivo phosphorus-31 studies of normal and cardiomyopathic myocardium. Magn Reson Med 1992;25:260-72. [Crossref] [PubMed]
- Ingwall JS. The hypertrophied myocardium accumulates the MB-creatine kinase isozyme. Eur Heart J 1984;5:129-39. [Crossref] [PubMed]
- Ingwall JS, Weiss RG. Is the Failing Heart Energy Starved?: On Using Chemical Energy to Support Cardiac Function. Circ Res 2004;95:135-45. [Crossref] [PubMed]
- Ten Hove M, Neubauer S. MR spectroscopy in heart failure--clinical and experimental findings. Heart Fail Rev 2007;12:48-57. [Crossref] [PubMed]
- Hudsmith LE, Neubauer S. Magnetic resonance spectroscopy in myocardial disease. JACC Cardiovascular imaging 2009;2:87-96. [Crossref] [PubMed]
- Neubauer S. Cardiovascular Magnetic Resonance Spectroscopy. In: Manning WJ, Pennell DJ, editors. Cardiovascular magnetic resonance. Elsevier Health Sciences, 2010:556-68.
- Holloway CJ, Suttie J, Dass S, et al. Clinical Cardiac Magnetic Resonance Spectroscopy. Prog Cardiovasc Dis 2011;54:320-7. [Crossref] [PubMed]
- Ventura-Clapier R, Garnier A, Veksler V, et al. Bioenergetics of the failing heart. Biochim Biophys Acta 2011;1813:1360-72. [Crossref] [PubMed]
- Peterson LR, Schilling J, Taegtmeyer H. Alterations in Cardiac Metabolism. In: Mann DL, Felker MG, editors. Heart failure: a companion to Braunwald's heart disease. Elsevier Health Sciences, 2014:254-71.
- Bottomley PA. MRS Studies of Creatine Kinase Metabolism in Human Heart. eMagRes 2016;5:1183-202.
- Peterzan MA, Lygate CA, Neubauer S, Rider OJ. Metabolic remodeling in hypertrophied and failing myocardium: a review. Am J Physiol Heart Circ Physiol 2017;313:H597-616. [Crossref] [PubMed]
- Hamman BL, Bittl JA, Jacobus WE, et al. Inhibition of the creatine kinase reaction decreases the contractile reserve of isolated rat hearts. Am J Physiol 1995;269:H1030-6. [PubMed]
- Tian R, Ingwall JS. Energetic basis for reduced contractile reserve in isolated rat hearts. Am J Physiol 1996;270:H1207-16. [PubMed]
- Tian R, Nascimben L, Kaddurah-Daouk R, et al. Depletion of Energy Reserve via the Creatine Kinase Reaction During the Evolution of Heart Failure in Cardiomyopathic Hamsters. J Mol Cell Cardiol 1996;28:755-65. [Crossref] [PubMed]
- Saks V, Dzeja P, Schlattner U, et al. Cardiac system bioenergetics: metabolic basis of the Frank-Starling law. J Physiol 2006;571:253-73. [Crossref] [PubMed]
- Hettling H, van Beek JH. Analyzing the Functional Properties of the Creatine Kinase System with Multiscale `Sloppy' Modeling. PLoS Comput Biol 2011;7:e1002130. [Crossref] [PubMed]
- Weiss RG, Gerstenblith G, Bottomley PA. ATP flux through creatine kinase in the normal, stressed, and failing human heart. Proc Natl Acad Sci U S A 2005;102:808-13. [Crossref] [PubMed]
- Gabr RE, El-Sharkawy AMM, Schär M, et al. Cardiac work is related to creatine kinase energy supply in human heart failure: a cardiovascular magnetic resonance spectroscopy study. J Cardiovasc Magn Reson 2018;20:81. [Crossref] [PubMed]
- Garnier A, Zoll J, Fortin D, et al. Control by Circulating Factors of Mitochondrial Function and Transcription Cascade in Heart Failure: A Role for Endothelin-1 and Angiotensin II. Circ Heart Fail 2009;2:342-50. [Crossref] [PubMed]
- Nascimben L, Ingwall JS, Pauletto P, et al. Creatine Kinase System in Failing and Nonfailing Human Myocardium. Circulation 1996;94:1894-901. [Crossref] [PubMed]
- Park SJ, Zhang J, Ye Y, et al. Myocardial creatine kinase expression after left ventricular assist device support. J Am Coll Cardiol 2002;39:1773-9. [Crossref] [PubMed]
- Sylvén C, Lin L, Jansson E, et al. Ventricular adenine nucleotide translocator mRNA is upregulated in dilated cardiomyopathy. Cardiovasc Res 1993;27:1295-9. [Crossref] [PubMed]
- Ingwall JS, Kramer MF, Fifer MA, et al. The Creatine Kinase System in Normal and Diseased Human Myocardium. N Engl J Med 1985;313:1050-4. [Crossref] [PubMed]
- Wu F, Zhang EY, Zhang J, et al. Phosphate metabolite concentrations and ATP hydrolysis potential in normal and ischaemic hearts. J Physiol 2008;586:4193-208. [Crossref] [PubMed]
- Bose S, French S, Evans FJ, et al. Metabolic network control of oxidative phosphorylation. Multiple roles of inorganic phosphate. J Biol Chem 2003;278:39155-65. [Crossref] [PubMed]
- Hollis DP, Nunnally RL, Taylor GJ IV, et al. Phosphorus nuclear magnetic resonance studies of heart physiology. J Magn Reson 1969;1978:319-30.
- Zhang J, Ishibashi Y, Zhang Y, et al. Myocardial bioenergetics during acute hibernation. Am J Physiol 1997;273:H1452-63. [PubMed]
- Cave AC, Ingwall JS, Friedrich J, et al. ATP Synthesis During Low-Flow Ischemia: Influence of Increased Glycolytic Substrate. Circulation 2000;101:2090-6. [Crossref] [PubMed]
- Martin C, Schulz R, Rose J, et al. Inorganic phosphate content and free energy change of ATP hydrolysis in regional short-term hibernating myocardium. Cardiovasc Res 1998;39:318-26. [Crossref] [PubMed]
- Valkovič L, Clarke WT, Schmid AI, et al. Measuring inorganic phosphate and intracellular pH in the healthy and hypertrophic cardiomyopathy hearts by in vivo 7T 31P-cardiovascular magnetic resonance spectroscopy. J Cardiovasc Magn Reson 2019;21:19. [Crossref] [PubMed]
- Jung WI, Sieverding L, Breuer J, et al. 31P NMR Spectroscopy Detects Metabolic Abnormalities in Asymptomatic Patients With Hypertrophic Cardiomyopathy. Circulation 1998;97:2536-42. [Crossref] [PubMed]
- Sieverding L, Jung WI, Breuer J, et al. Proton-Decoupled Myocardial 31P NMR Spectroscopy Reveals Decreased PCr/Pi in Patients with Severe Hypertrophic Cardiomyopathy. Am J Cardiol 1997;80:34A-40A. [Crossref] [PubMed]
- Middeldorp ME, Pathak RK, Meredith M, et al. PREVEntion and regReSsive effect of weight-loss and risk factor modification on Atrial Fibrillation: The REVERSE-AF study. Europace 2018;20:1929-35. [Crossref] [PubMed]
- Mudd JO, Kass DA. Tackling heart failure in the twenty-first century. Nature 2008;451:919-28. [Crossref] [PubMed]
- Gupta A, Akki A, Wang Y, et al. Creatine kinase-mediated improvement of function in failing mouse hearts provides causal evidence the failing heart is energy starved. J Clin Invest 2012;122:291-302. [Crossref] [PubMed]
- Gupta A, Rohlfsen C, Leppo MK, et al. Creatine Kinase-Overexpression Improves Myocardial Energetics, Contractile Dysfunction and Survival in Murine Doxorubicin Cardiotoxicity. PLoS One 2013;8:e74675. [Crossref] [PubMed]
- Zervou S, Whittington HJ, Ostrowski PJ, et al. Increasing creatine kinase activity protects against hypoxia / reoxygenation injury but not against anthracycline toxicity in vitro. PLoS ONE 2017;12:e0182994. [Crossref] [PubMed]
- Whittington HJ, Ostrowski PJ, McAndrew DJ, et al. Over-expression of mitochondrial creatine kinase in the murine heart improves functional recovery and protects against injury following ischaemia-reperfusion. Cardiovasc Res 2018;114:858-69. [Crossref] [PubMed]
- Lygate CA, Bohl S, Ten Hove M, et al. Moderate elevation of intracellular creatine by targeting the creatine transporter protects mice from acute myocardial infarction. Cardiovasc Res 2012;96:466-75. [Crossref] [PubMed]
- Whittington HJ, McAndrew DJ, Cross RL, et al. Protective effect of creatine elevation against ischaemia reperfusion injury is retained in the presence of co-morbidities and during cardioplegia. PLoS One 2016;11:e0146429. [Crossref] [PubMed]
- Lygate CA, Medway DJ, Ostrowski PJ, et al. Chronic creatine kinase deficiency eventually leads to congestive heart failure, but severity is dependent on genetic background, gender and age. Basic Res Cardiol 2012;107:276. [Crossref] [PubMed]
- Oudman I, Clark JF, Brewster LM. The Effect of the Creatine Analogue Beta-guanidinopropionic Acid on Energy Metabolism: A Systematic Review. PLoS One 2013;8:e52879. [Crossref] [PubMed]
- Hirsch GA, Bottomley PA, Gerstenblith G, et al. Allopurinol acutely increases adenosine triphospate energy delivery in failing human hearts. J Am Coll Cardiol 2012;59:802-8. [Crossref] [PubMed]